Posts by Align Manufacturing
Trump Tariffs and Their Implications
Tariff Proposals:
Trump aims to impose broad tariffs to address trade imbalances and boost U.S. manufacturing.
Proposes creating a new agency, the “External Revenue Service,” to collect tariffs directly from foreign importers.
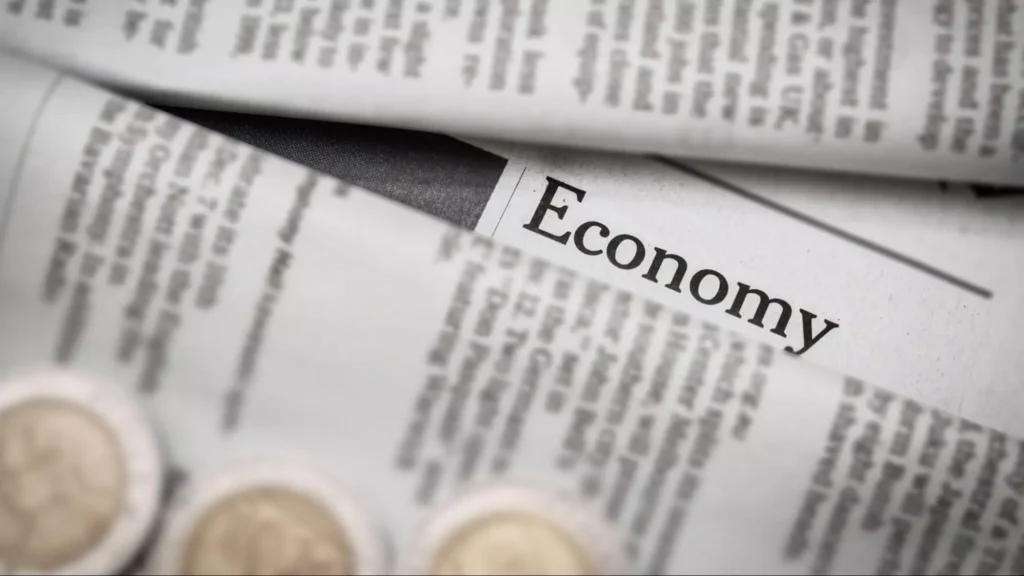
Impact on Trade and Economy:
Short-Term Costs:
Tariffs would increase prices for U.S. consumers, affecting essential goods like apparel, appliances, and toys.
Organizations like the National Retail Federation warn of a reduction in consumer spending power by up to $78 billion.
Long-Term Adjustments:
Tariffs may lead to restructuring of global supply chains, but the U.S. might remain too expensive for onshore manufacturing.
Historical Context:
Under Trump’s previous administration, tariffs on $360 billion worth of Chinese goods triggered a trade war.
Subsequent actions by President Biden retained and expanded tariffs, highlighting bipartisan support for economic protectionism.
Strategic Considerations:
While tariffs aim to boost U.S. jobs and revenue, they risk economic retaliation and higher costs for businesses and consumers.
Critics argue that such measures create a “lose-lose” scenario: exporters face reduced demand, while U.S. consumers and businesses bear higher costs.
Global Trends:
The rise in tariffs reflects a broader trend of linking economic security to national security.
Countries are focusing on industrial policies to ensure self-reliance and mitigate geopolitical risks.
China’s Resilience:
Despite tariffs, China’s cost-efficient manufacturing capabilities continue to dominate global markets.
Tariffs often lead to indirect imports via other countries, circumventing their intended effects.
Interesting Insights
Tariffs might generate short-term government revenue but risk eroding consumer spending and global economic growth.
Biden, despite criticizing tariffs during his campaign, upheld many of Trump’s trade policies, illustrating a global shift toward protectionism.
Manufacturing and services are becoming intertwined as nations prioritize self-reliance in critical industries.
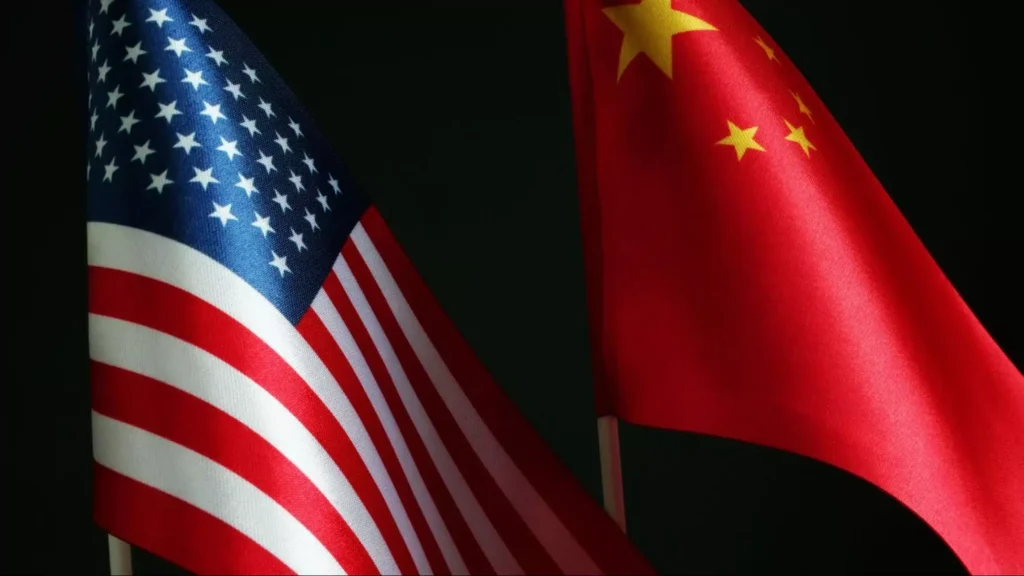
Watch more here
Trump Tariffs and Their Implications
The U.S. President-elect Donald Trump’s proposed tariffs, including a 10% universal import tariff and rates as high as 60% on Chinese goods, present a unique challenge for businesses dependent on international supply chains. These tariffs are aimed at addressing trade imbalances and promoting U.S. manufacturing, but they also significantly increase costs for businesses and consumers alike. For companies navigating these changes, Align Manufacturing offers a solution to ensure supply chain stability, cost efficiency, and resilience in a volatile global trade environment.
1. Mitigating Tariff Impact
- Align Manufacturing provides access to a diversified manufacturing network in Southeast Asia (SEA), including countries like Vietnam, Thailand, and India. By shifting production to these regions, businesses can significantly reduce exposure to high tariffs on Chinese goods.
- SEA’s favorable trade agreements with the U.S. and other markets offer additional cost savings and competitive advantages.
2. Cost-Effective Solutions
- Align Manufacturing’s partners in SEA provide competitive labor and production costs without compromising on quality. This ensures that businesses can maintain profitability even amidst rising tariffs.
- Our expertise in sourcing the right manufacturing processes ensures efficient production tailored to your specific needs.
3. Access to High-Quality Manufacturing
- With decades of experience, Align Manufacturing ensures high standards across all projects, leveraging advanced technologies and robust quality control measures.
- We collaborate with reliable SEA manufacturers who are investing in quality infrastructure and sustainable practices.
4. Supply Chain Resilience
- Diversifying your manufacturing base with Align Manufacturing reduces reliance on a single source, such as China, and minimizes disruptions caused by trade wars or geopolitical instability.
- Our global expertise ensures smooth operations and on-time delivery, even in challenging trade environments.
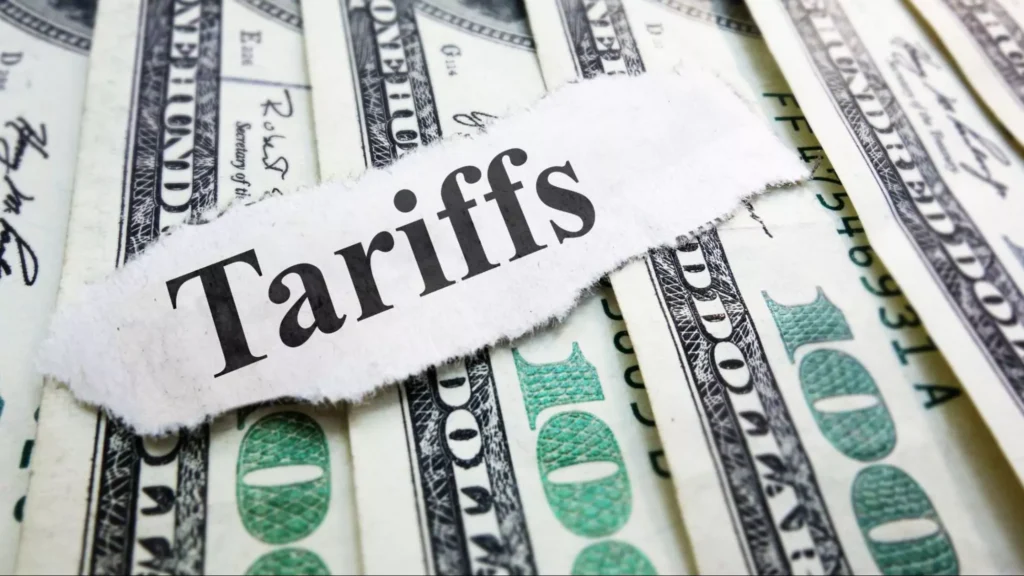
The Strategic Advantage of Align Manufacturing
The proposed tariffs highlight the importance of proactive supply chain management and diversification. Align Manufacturing is uniquely positioned to help businesses transition smoothly, offering:
- Tailored Manufacturing Solutions: We customize our services to fit your product requirements, ensuring cost-effective and high-quality outcomes.
- Comprehensive Support: From initial consultation to final delivery, Align Manufacturing offers end-to-end support, ensuring your operations remain seamless.
- Trusted Expertise: Our deep knowledge of SEA markets and long-standing relationships with manufacturers provide businesses with a competitive edge.
Why Now is the Time to Act
Trump’s tariff proposals underscore the urgency for businesses to reevaluate their global manufacturing strategies. Delaying action could result in higher costs, supply chain disruptions, and lost market share. By partnering with Align Manufacturing, businesses can:
- Avoid the financial strain of tariffs by leveraging cost-effective SEA manufacturing.
- Maintain product quality and delivery timelines with a diversified supply chain.
- Stay competitive in an increasingly protectionist global market.
Conclusion
Align Manufacturing is your strategic partner in navigating the complexities of global trade. With our expertise in Southeast Asia’s manufacturing landscape, we help businesses reduce costs, mitigate risks, and thrive despite tariff challenges.
Contact Align Manufacturing today to learn how we can support your business in adapting to these changing trade dynamics and securing a competitive advantage.
Visit Align Manufacturing and explore our manufacturing services to learn more about how we can help your business succeed in the face of global trade challenges.
What is Gravity Die Casting?
Gravity die casting is a metal casting technique that uses gravity rather than external pressure to fill a mold. In this process, molten aluminum is poured into a steel or iron mold, known as a "die," where it flows down into the mold cavity under the force of gravity. This method differs from other types of casting, such as high-pressure die casting, as it relies solely on the natural pull of gravity, resulting in less turbulent flow and fewer air pockets. The result is a dense, high-quality metal part with excellent dimensional accuracy and strength, making it especially suitable for components requiring durability and precision.
The process achieves tolerances of ±0.3mm to ±0.5mm, with wall thickness capabilities ranging from 3mm to 20mm. Parts can range from small components weighing 0.1 kg to larger pieces up to 100 kg, offering remarkable versatility in production capabilities.
Compared to sand casting, gravity die casting is more efficient in terms of both material use and production time. The reusable steel or iron molds used in gravity casting allow for rapid production cycles and consistent quality across batches, making it a cost-effective solution for medium-to-high volume production runs.Its ability to produce parts in quantities ranging from 250 to 50,000 units per annum makes it a popular choice.This adaptability is achieved through its efficient use of resources and streamlined production cycles.
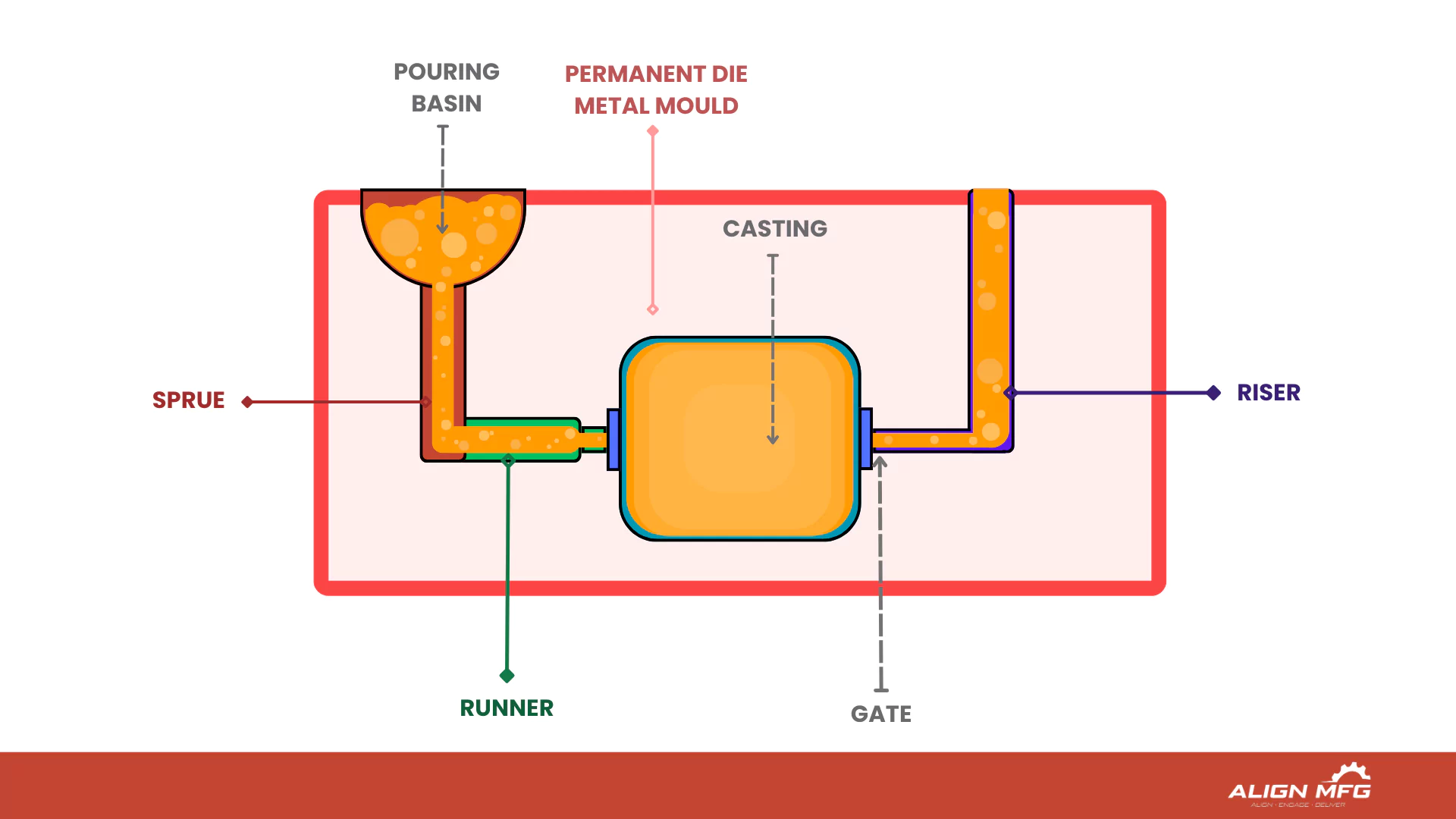
Process of Aluminum Gravity Die Casting
The aluminum gravity die casting process is straightforward yet precise, requiring careful handling and skilled workmanship to ensure optimal results. Here’s a step-by-step breakdown of how the process works:
1.Preparing the Mold
The process begins with preheating the die to temperatures between 200-300°C to prevent thermal shock and ensure smooth flow of molten aluminum, which is typically heated to 650-750°C. Lubricants are applied to the mold to help with release after solidification. The mold design incorporates specific features like draft angles of 2-3° for external surfaces and strategically placed parting lines to ensure optimal results."
2.Pouring the Molten Aluminum
Once the mold is ready, molten aluminum is poured into the mold cavity. As gravity pulls the molten metal into every corner of the mold, it begins to fill the cavity, shaping the metal according to the mold’s design. This step requires precise control to ensure an even flow and avoid air pockets.
3.Solidification and Cooling
After pouring, the aluminum is left to solidify within the mold. The cooling phase is crucial, as controlled cooling can impact the material's final properties, such as its strength and structural integrity. Faster cooling generally produces finer grain structures, which can enhance the material's durability.
4.Removing the Casting
Once the metal has solidified and cooled to a manageable temperature, the mold is opened, and the casting is removed. Any residual material, such as sprues or runners, is trimmed off in preparation for the finishing process.
5.Finishing and Inspection
The final casting may go through secondary operations like machining, polishing, or surface treatments to achieve the desired finish and precision. Each part undergoes inspection to ensure it meets quality standards and specifications before moving on to the next phase.
Advantages of Aluminum Gravity Die Casting
Aluminum gravity die casting offers several distinct advantages that make it an attractive choice for various industries. Here are some of the key benefits:
- High Dimensional Accuracy
Gravity die casting produces components with precise dimensions and excellent surface finishes, reducing the need for additional machining. This accuracy is ideal for parts that must meet tight tolerances and specifications.Compared to alumnium sand casting - Superior Strength and Density
Quality control is paramount in gravity die casting. The process includes comprehensive testing methods such as X-ray inspection, pressure testing, and dimensional verification to prevent common defects like porosity and shrinkage. Surface finishing options range from as-cast finish to various post-processing treatments including machining, coating, and painting, depending on the application requirements. - Cost-Effective for Medium-to-High Production Volumes
The reusable nature of steel or iron molds makes gravity die casting cost-effective for medium-to-high production runs. The upfront investment in the mold is offset by the long-term savings from its reusability and the consistency it provides. - Enhanced Mechanical Properties
Components produced by gravity die casting generally exhibit better mechanical properties compared to sand-cast parts, thanks to the controlled cooling and reduced air entrapment. These properties make gravity die-cast parts suitable for applications where durability and longevity are critical. - Eco-Friendly and Less Material Waste
Gravity die casting tends to produce less material waste than sand casting, as the molds can be reused multiple times. The efficiency of the process also reduces the need for excess metal, making it more environmentally friendly. - Improved Production Speed
The rapid cooling time of metal molds in gravity die casting allows for faster production cycles, making it a practical choice for projects needing quick turnarounds without sacrificing quality.
Aluminum Gravity Die Casting Applications
Aluminum gravity die casting is widely used across various industries, especially in sectors where precision, strength, and reliability are paramount. Some of the common applications include:
- Automotive Industry
Gravity die casting is often used to create engine components, transmission cases, and suspension parts. Aluminum alloy A357 is particularly favored for its higher strength properties, while A356 is chosen for parts requiring excellent fluidity and corrosion resistance. The process's ability to maintain uniform wall thickness and proper corner radii makes it ideal for complex automotive components. - Aerospace Industry
Aerospace manufacturers use gravity die casting for parts that require excellent mechanical properties and corrosion resistance. Components like housings, brackets, and supports benefit from the dimensional accuracy and structural integrity of gravity-cast aluminum. - Industrial Machinery
Many machinery parts, including hydraulic components, pump housings, and gearboxes, are produced using gravity die casting. Using alloys like A356 for its excellent fluidity and corrosion resistance, these components can be manufactured with wall thicknesses ranging from 3mm to 20mm. The process ensures uniform material properties through controlled cooling and proper mold design, incorporating features like strategic parting lines and adequate draft angles (2-3°) for easy part removal. - Consumer Electronics and Appliances
Aluminum gravity die casting is also common in consumer electronics and home appliances, where it’s used to create durable, lightweight casings and structural parts. The method’s accuracy and finish quality are ideal for products with aesthetic and functional requirements. - Medical Equipment
Medical devices and equipment that require reliable, corrosion-resistant parts often incorporate gravity die-cast aluminum components. From casings to support structures, gravity die casting ensures that medical equipment meets high standards for both safety and durability. - Marine Industry
Due to aluminum’s natural corrosion resistance and strength, gravity die-cast aluminum parts are widely used in marine applications such as motor housings, brackets, and propeller blades. These components benefit from the durability and resistance to seawater corrosion that gravity casting provides.
Types of components
Gravity Die Casting (GDC), which is typically used for producing medium to high-precision parts with moderate complexity, here are the relevant components that Align Manufacturing could produce using GDC:
1. Oil & Gas Industry Components
- Flanges: High-strength parts used to connect pipes and equipment, benefiting from the precision and consistency of GDC.
- Valve Bodies: Ideal for controlling fluid flow, requiring dimensional accuracy and durability.
- Impellers: Used in pumps for fluid movement, made with fine surface finishes and low porosity offered by GDC.
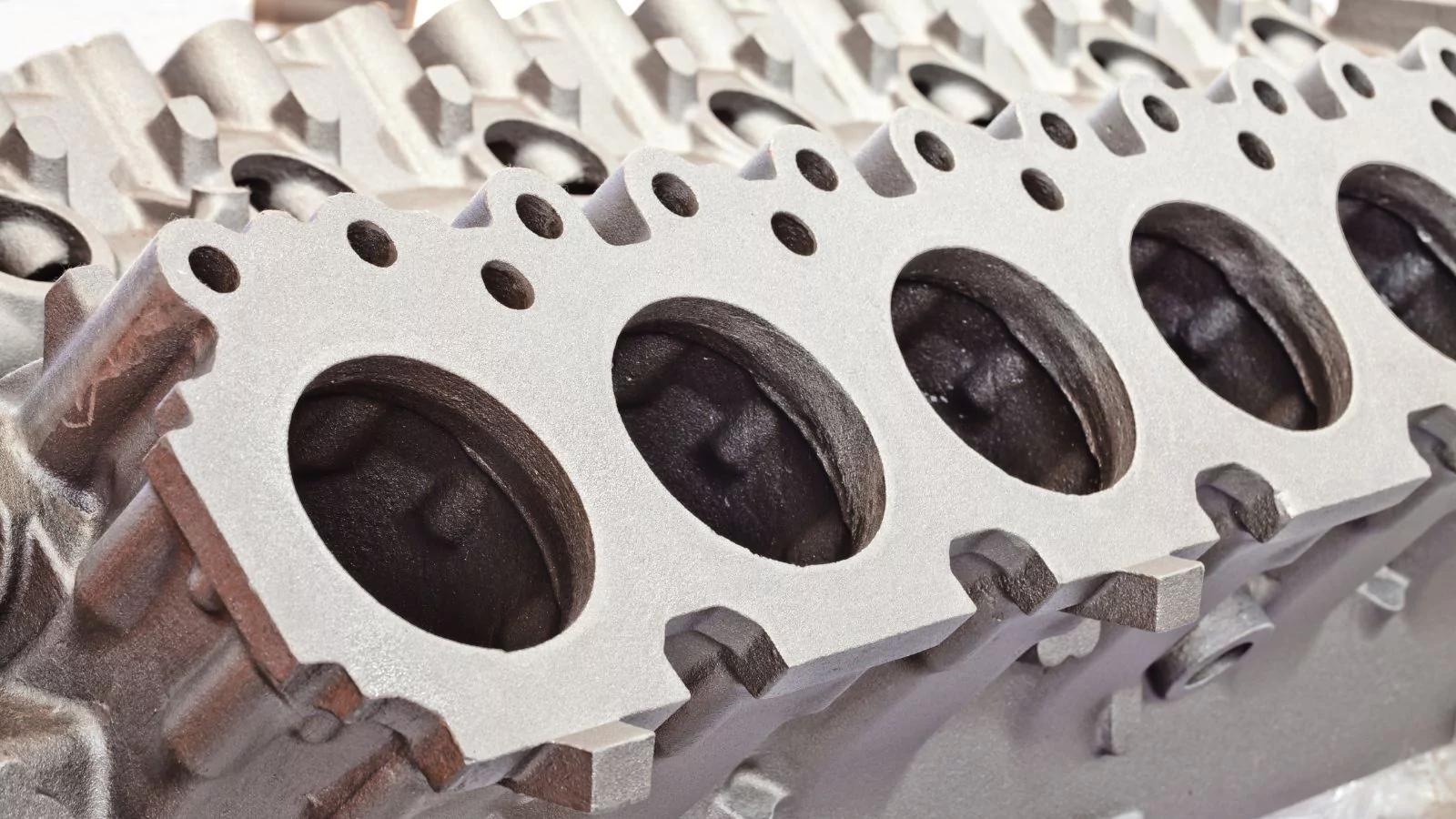
2. Truck & Trailer Industry Components
- Suspension Parts: Durable components benefiting from GDC's ability to produce parts with high structural integrity.
- Engine Brackets: Precision-engineered to securely mount engines while reducing vibrations.
- Brake Rotors: Made with precise dimensions and excellent surface finishes for consistent braking performance.
- Hubs: Cast with high accuracy to support smooth wheel rotation.
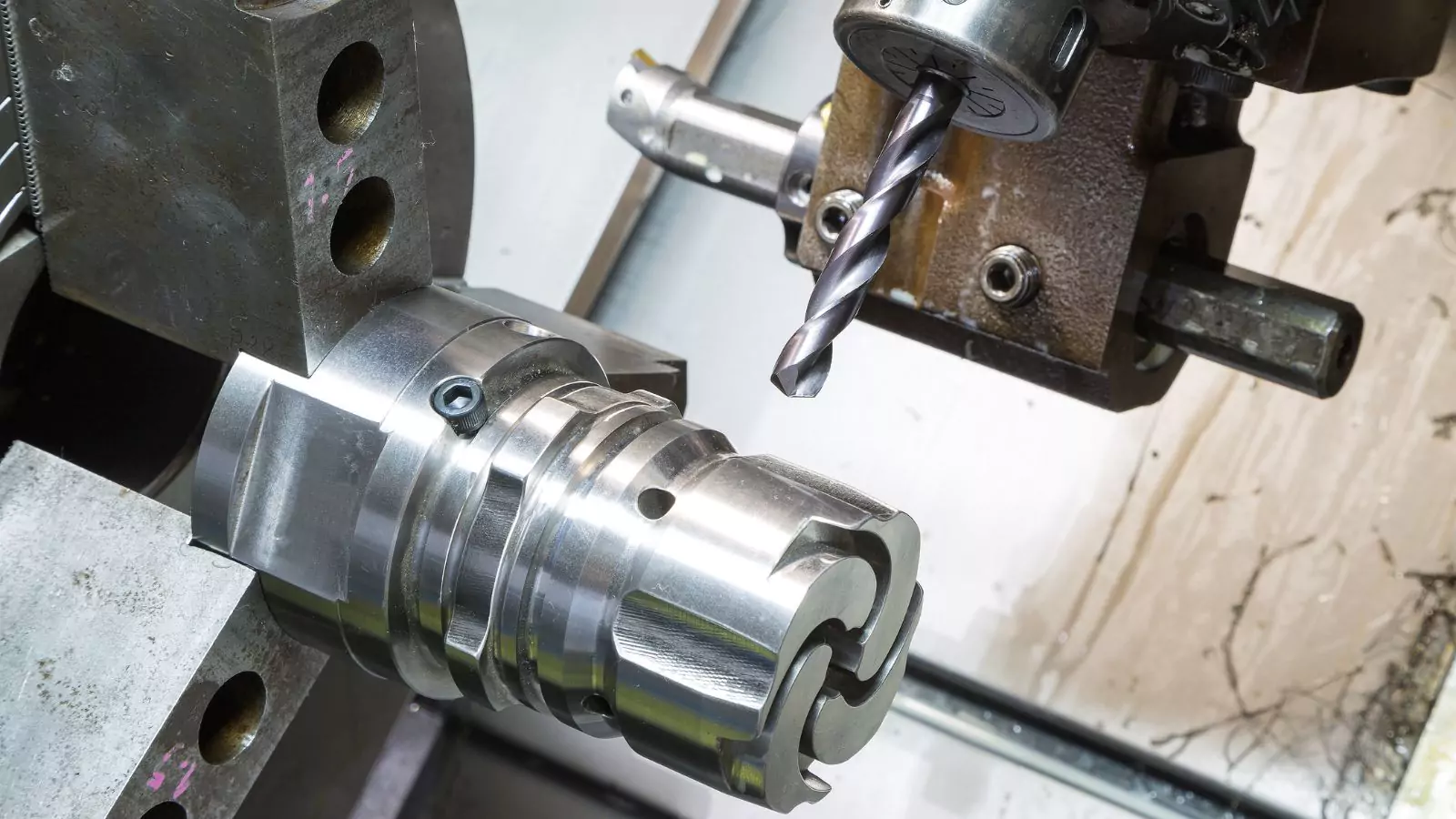
- Industrial Components
- Manhole Covers: High-strength covers that can be precisely cast for consistent performance.
- Vent Release Valves: Suitable for precise control of gases or liquids, taking advantage of GDC’s low porosity properties.
Best Practices and Quality Control
- Mold Design Optimization:
- Proper gating system design for smooth metal flow
- Strategic placement of cooling channels
- Adequate venting to prevent gas entrapment
- Draft angles of 2-3° minimum for external surfaces
- Temperature Management:
- Die preheating to 200-300°C
- Maintaining consistent pouring temperature (650-750°C)
- Controlled cooling rates for optimal material properties
- Quality Assurance:
- X-ray inspection for internal defects
- Pressure testing for leakage
- Dimensional verification using precision measurement tools
- Surface finish inspection and testing
- Surface Finishing Options:
- As-cast finish for non-critical surfaces
- Machining for precise dimensional requirements
- Various coating options for enhanced durability
- Paint preparation and application when required
Conclusion
Aluminum gravity die casting remains a vital process for producing high-quality, precise components across various industries. Its unique combination of strength, lightweight properties, and cost-effectiveness make it ideal for applications ranging from automotive to medical equipment. By using gravity to guide molten metal into molds, this casting method achieves impressive dimensional accuracy and surface finish, meeting the needs of industries that demand reliability and durability.
If you’re interested in learning more about other casting methods and how they compare, explore the wide range of casting processes available on Align Manufacturing’s website. Each method offers unique benefits tailored to specific applications, helping you find the perfect solution for your manufacturing needs.
FAQs on Gravity Die Casting
Gravity die casting primarily uses non-ferrous metals, with aluminum alloys being the most common. Specific alloys include:
- A356: Excellent for general-purpose applications, offering good fluidity and corrosion resistance
- A357: Higher strength applications requiring superior mechanical properties
- Other materials include zinc alloys, magnesium alloys, and some copper-based alloys Each material requires specific die temperatures and pouring temperatures for optimal results."
Gravity die casting offers distinct advantages in terms of precision (±0.3mm to ±0.5mm tolerances) compared to sand casting, though it may be slower than high-pressure die casting. The initial tooling cost is higher than sand casting but lower than high-pressure die casting. Part size capabilities (up to 100 kg) and production volumes (250-50,000 units annually) position it perfectly for medium-to-high volume production runs."
Manual Process Time: While manual gravity die casting offers superior precision (±0.3mm tolerance), it requires longer production cycles compared to high-pressure die casting.
Design Restrictions: Complex geometries with deep recesses or intricate internal features can be challenging to produce, as the process relies on natural metal flow. Wall thickness must be carefully controlled between 3-20mm for optimal results.
Initial Investment: Tooling costs are significant due to the need for high-quality steel molds designed with proper gating systems, cooling channels, and venting.
Temperature Management: Precise control of both die temperature (200-300°C) and molten metal temperature (650-750°C) is crucial for quality, adding complexity to the process.
Industries that require lightweight, high-strength components benefit greatly from aluminum gravity die casting. These include the automotive, aerospace, industrial machinery, consumer electronics, medical equipment, and marine industries. Each of these sectors relies on the process to produce parts that are durable, corrosion-resistant, and capable of meeting stringent performance standards.
Gravity die casting is relatively sustainable compared to other casting methods. The reusable molds minimize waste, and the aluminum used is recyclable, reducing the environmental impact. Additionally, the process’s efficiency in terms of material usage and energy consumption contributes to its eco-friendliness. Many manufacturers prioritize recycling aluminum and repurposing materials to make the process even more sustainable.
Friend-shoring, Near-shoring, Reshoring, and Other Buzzwords
Supply chain landscape is evolving rapidly, fueled by shifting geopolitics, emerging technologies, and changing consumer demands. Terms like friend-shoring, near-shoring, and re-shoring are gaining traction as businesses seek to adapt. But what do these buzzwords mean, and how do they impact global supply chain strategies?
In this article, we'll explore these key concepts, compare their benefits, challenges, and applications, and provide actionable insights for supply chain professionals. By the end, you'll understand why companies like Align Manufacturing are leading the way in navigating these trends.
What is Friend-shoring?
Friend-shoring is the strategic relocation of supply chains to countries that share aligned political, economic, or ideological interests. This emerging approach addresses growing concerns over geopolitical risks, fostering economic collaboration among nations that are considered reliable allies.
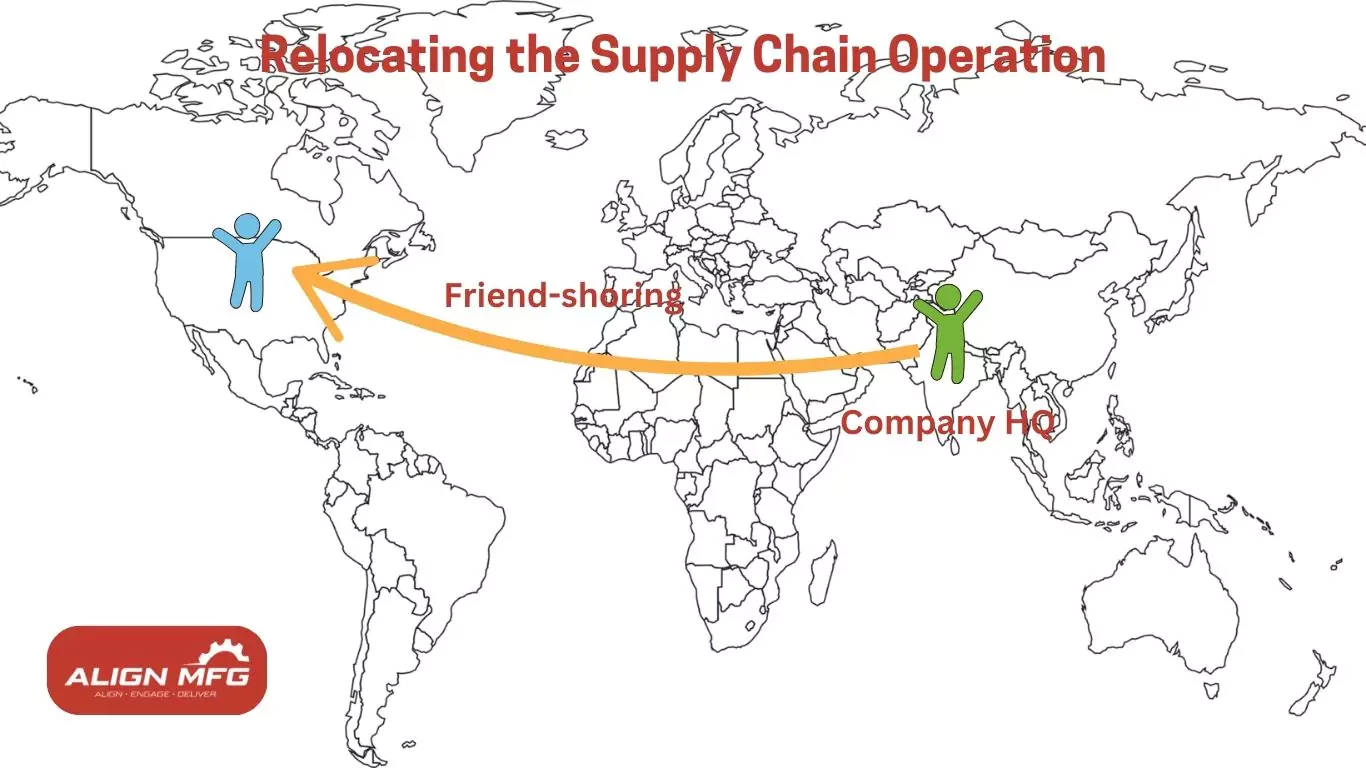
Key Objectives of Friend-shoring:
- Mitigating Risks: By focusing on countries with stable governance and aligned values, businesses minimize the risk of trade disruptions caused by geopolitical tensions.
- Strengthening Alliances: Encourages mutual economic growth and reinforces trust among allied nations.
- Reducing Overdependence: Diversifies supply chains, reducing reliance on politically unstable or adversarial countries.
Benefits of Friend-shoring:
- Reduced Geopolitical Risks: Relocating supply chains to trusted countries ensures continuity even during global tensions.
- Improved Economic Collaboration: Aligning trade policies and practices fosters smoother operations and better partnerships.
- Examples in Action: Semiconductor manufacturers are a notable example, shifting production to countries within alliances like NATO to reduce dependency on regions with volatile relations, such as China.
Challenges of Friend-shoring:
- Finding Reliable Partners: Evaluating a nation’s infrastructure, workforce skills, and regulatory environment is critical.
- Regulatory Variations: Even allied countries may have differing legal frameworks, complicating compliance.
- Cost Implications: While offering political stability, these shifts may incur higher costs compared to traditional offshoring.
Friend-shoring is gaining momentum as nations and businesses aim to build resilient supply chains in the face of geopolitical instability. Its focus on allied nations offers a pragmatic solution for long-term sustainability.
Exploring Near-shoring
Near-shoring refers to the relocation of supply chain operations closer to a company’s home market. This strategy improves operational efficiency by reducing transportation costs and delivery times, offering an agile response to market demands.
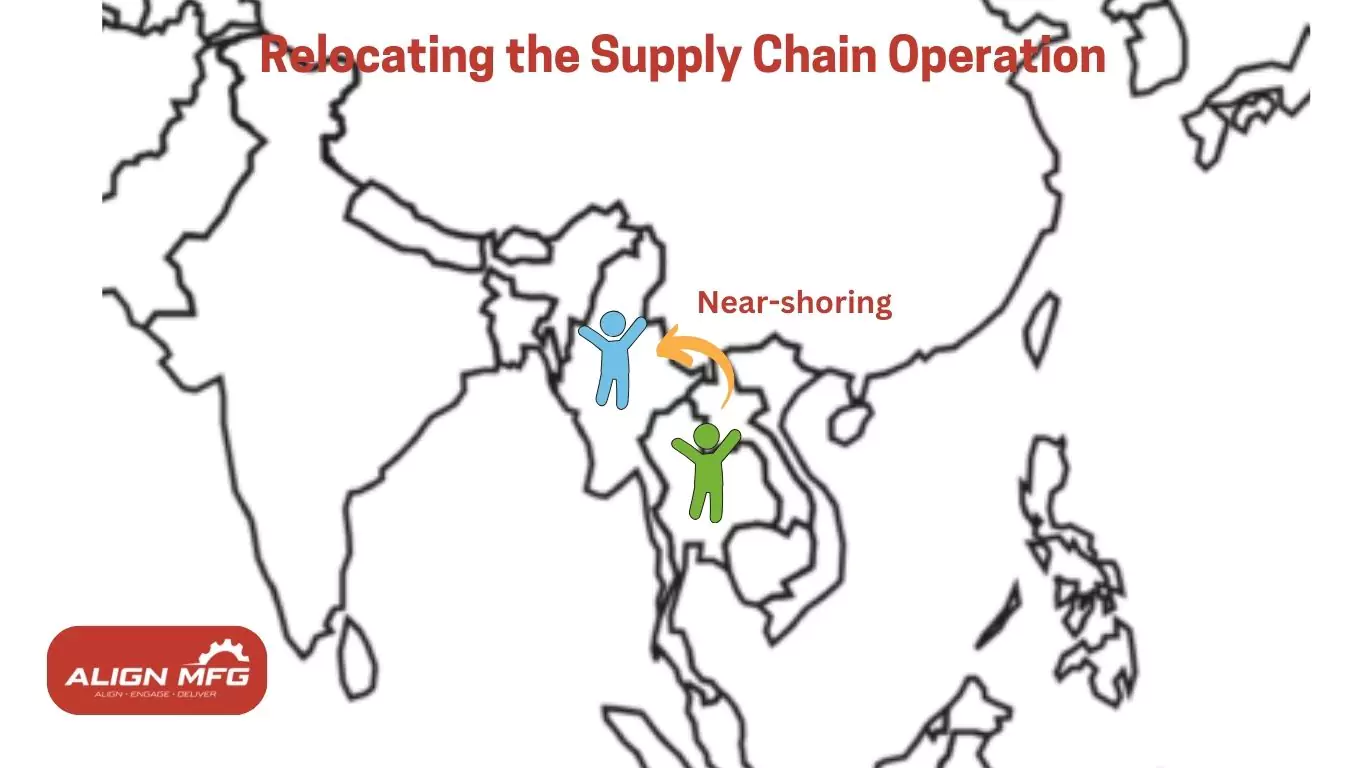
Core Principles of Near-shoring:
- Proximity: Operating closer to key markets reduces time-to-market.
- Cost Efficiency: While not as low-cost as offshoring, reduced logistics expenses offset higher labor costs.
- Supply Chain Agility: Faster access to resources and production facilities enhances adaptability.
Benefits of Near-shoring:
- Faster Delivery Times: Shortened supply chains mean quicker access to products for consumers.
- Reduced Transportation Costs: Geographic proximity lowers shipping and storage expenses.
- Enhanced Collaboration: Being closer geographically often fosters better communication and understanding with suppliers.
Real-World Applications:
- Example: Many U.S. companies are opting to shift operations to Mexico rather than Asia to save on costs and reduce transit delays.
- Case Study: A North American electronics manufacturer reported a 40% decrease in delivery times after relocating operations to Mexico, allowing them to better compete with local rivals.
Challenges of Near-shoring:
- Labor Costs: Though lower than home markets, labor costs are often higher than traditional offshoring destinations.
- Infrastructure Readiness: Some near-shore countries may lack adequate infrastructure, leading to bottlenecks.
- Supplier Availability: Limited availability of specialized suppliers in certain regions can hinder progress.
Near-shoring is a middle-ground strategy, balancing operational proximity with cost efficiency, making it an attractive option for many industries.
Re-shoring: Bringing It Home
Re-shoring, also called onshoring, involves moving manufacturing or production processes back to the company’s home country. This strategy emphasizes boosting domestic economic activity, enhancing brand reputation, and reducing external dependencies.
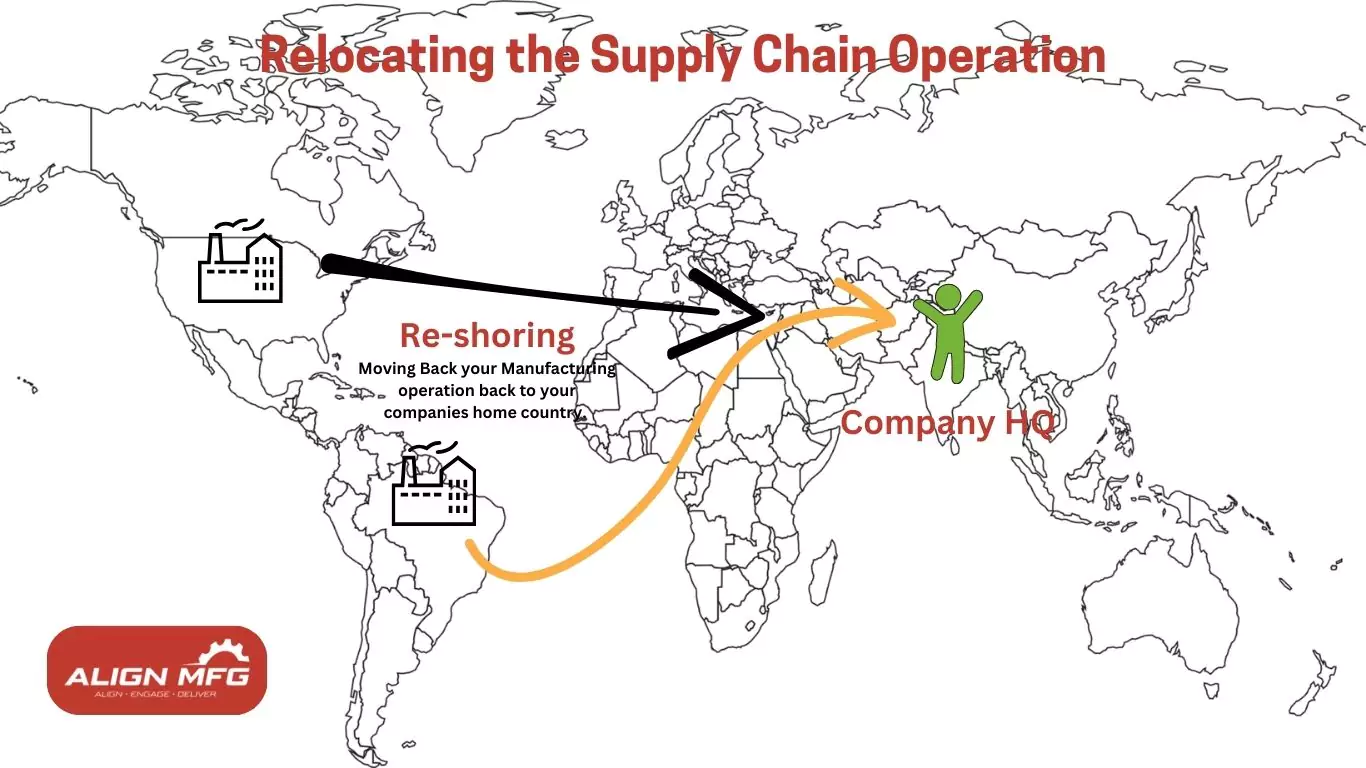
Why Companies are Re-shoring:
- Government Incentives: Many governments provide tax breaks, grants, or subsidies to encourage businesses to bring operations back home.
- Consumer Demand: “Made in the USA” or similar homegrown labels resonate strongly with consumers, fostering trust and brand loyalty.
- Supply Chain Stability: By operating domestically, businesses avoid global shipping uncertainties and reduce overreliance on external suppliers.
Benefits of Re-shoring:
- Job Creation: Brings employment opportunities back to domestic markets.
- Improved Quality Control: Having production closer allows for stringent oversight and quality assurance.
- Enhanced Resilience: Reduces the risk of disruptions caused by international supply chain interruptions.
Examples in Practice:
- Apple: While much of its manufacturing remains overseas, Apple has partially re-shored some production of key components to the U.S., ensuring compliance with local quality standards.
- Boeing: Relocated significant operations back to U.S. soil to address concerns over outsourcing quality and supply chain resilience.
Challenges of Re-shoring:
- Higher Costs: Domestic labor is generally more expensive compared to traditional offshore or near-shore options.
- Logistical Complexity: Transitioning existing supply chains to domestic locations involves significant planning and investment.
- Scaling Issues: Home markets may lack the resources or infrastructure to support large-scale operations in certain industries.
Re-shoring is often seen as a long-term investment, prioritizing quality, reliability, and local economic growth over immediate cost savings.
Offshoring: The Precursor
Offshoring refers to outsourcing production or services to countries with lower labor and operational costs. This practice, dominant in the late 20th century, was a cornerstone of globalization and remains a key strategy for cost reduction.
Why Offshoring Became Popular:
- Cost Savings: Access to cheaper labor markets and lower production costs made offshoring highly lucrative.
- Scalability: The ability to rapidly scale operations in manufacturing hubs like China or India.
- Global Markets: Many companies capitalized on both low production costs and new consumer markets in these regions.
Benefits of Offshoring:
- Significant Cost Reductions: Labor and operational expenses are substantially lower in many developing nations.
- Increased Capacity: Access to a larger, cost-effective workforce allows companies to expand rapidly.
- Global Reach: Establishing a presence in foreign markets often aligns with the growth of a global consumer base.
Current Challenges with Offshoring:
- Supply Chain Disruptions: Events like COVID-19 exposed vulnerabilities in relying on global supply chains.
- Reputation Concerns: Companies face criticism over ethical concerns, such as poor labor conditions in certain regions.
- Geopolitical Risks: Trade wars, sanctions, and international conflicts can severely impact operations.
While offshoring remains a vital strategy for many businesses, its drawbacks are causing companies to reconsider alternative approaches, such as near-shoring and friend-shoring, to mitigate risks and improve resilience.
Benefits of These Strategies
Strategy | Cost | Risk Mitigation | Delivery Speed |
Friend-shoring | Moderate | High | Moderate |
Near-shoring | High | High | High |
Re-shoring | Highest | Very High | Highest |
Current Trends in Supply Chain Strategies
Modern strategies emphasize regionalization, automation, and sustainability. For example:
- Digital twins for monitoring supply chains.
- Blockchain for transparency.
- Geopolitical events like Brexit are increasing regionalization efforts.
Future Predictions
The future of supply chain management will likely see:
- Increased investment in technology like AI for forecasting.
- Expansion of regional trade networks.
Role of Align Manufacturing in Supporting Strategies
Align Manufacturing offers expertise in simplifying these transitions by:
- Streamlining logistics through technology.
- Providing end-to-end supply chain solutions tailored to business needs.
Why Align Manufacturing is Your Partner
With its expertise in supply chain optimization, Align Manufacturing helps businesses stay ahead in a volatile global market. Their custom solutions, supported by cutting-edge technology, ensure smooth implementation of these strategies.
Conclusion
Adapting your supply chain strategy isn’t just a competitive advantage—it’s a survival necessity. Approaches like friend-shoring, near-shoring, and re-shoring enable businesses to enhance resilience, optimize costs, and strengthen their market positioning. However, each strategy comes with unique benefits and challenges, requiring careful evaluation to align with your business goals.
Companies like Align Manufacturing make navigating these transitions seamless, leveraging their expertise in supply chain optimization and cutting-edge technology to craft tailored solutions. Whether you’re mitigating risks, reducing delivery times, or enhancing supply chain agility, Align Manufacturing is your trusted partner for turning strategy into success.
FAQ
- Friend-shoring: Relocates operations to allied countries with shared political and economic values to minimize geopolitical risks.
- Near-shoring: Moves operations closer to the home market to reduce transportation costs and improve delivery times.
- Re-shoring: Brings manufacturing or production back to the company’s home country for improved quality control and local job creation.
Companies are prioritizing resilience and risk mitigation over cost savings. Friend-shoring and near-shoring reduce dependence on volatile regions, improve supply chain agility, and address concerns over geopolitical instability and supply chain disruptions exposed during events like COVID-19 and trade wars.
Re-shoring benefits include:
- For businesses: Improved quality control, reduced shipping uncertainties, and enhanced brand reputation with "Made in Home Country" labeling.
- For the economy: Boosts domestic employment, supports local industries, and promotes economic growth.
The decision depends on several factors:
- Cost considerations: Offshoring is often the cheapest but comes with higher risks.
- Proximity needs: Near-shoring suits businesses requiring quicker delivery times.
- Geopolitical concerns: Friend-shoring reduces risks by focusing on stable and allied regions.
- Brand reputation: Re-shoring appeals to consumers seeking local products.
A detailed cost-benefit analysis and risk assessment are critical before making a decision.
Industries that rely heavily on timely delivery, quality control, or geopolitical stability benefit the most, such as:
- Healthcare: Ensures quick delivery of medical supplies.
- Automotive: Reduces risks of disruptions in complex, multi-tier supply chains.
- Technology: Protects intellectual property by operating in trusted locations.
- Retail and E-commerce: Improves delivery speed to consumers by relocating closer to home markets.
Why Manufacturers Need to Get Out of China Now: Strategic Insights from Align Manufacturing
In this video, Casey Ross and Benjamin Unruh from Align Manufacturing and Alex Chiu from Maesot Heavy Industries discuss why manufacturers must urgently consider moving their operations out of China. They explore the impact of geopolitical tensions, rising tariffs, and the key strategies for ensuring a smooth transition to Southeast and South Asia.
Casey: Hi everyone, I'm Casey Ross, Partner at Align Manufacturing. I'm here today with Benjamin Unruh, Partner at Align Manufacturing, and Alex Chiu, Deputy General Manager at Maesot Heavy Industries, to discuss the critical shifts in global manufacturing landscapes and the strategic importance of relocating production from China to more favorable markets in Southeast and South Asia. Ben, Alex, thank you for joining us here on this podcast. Alex, why don't you introduce yourself? You have a very interesting background. So please, tell us about your background and also a little bit about your business.
Alex: Hi. Thank you, Casey and Ben for having me in this podcast. My name is Alex. I'm from Maesot Heavy Industries. Um, we are actually a family owned business. We predominantly do foundry and sand casting, furan resin, green sand and shell molding. I actually graduated from the US with a bachelors in mechanical engineering. We have been in Thailand for a bit more than I would say 8 to 9 years. Yeah. And, we work with many different companies, including foreign like North American companies, Japanese companies, and of course the local Thai industries. Right.
Casey: And I think you actually, you have a really interesting background because being Taiwanese and living in Thailand, owning a factory here gives you a very interesting perspective on what we were going to discuss in this podcast. So yeah, thank you for joining us. So, let's let's dive into it. What is driving the necessity for OEMs to get out of China, or at least have a China plus one?
Ben: Yeah, I mean, the the big one on everyone's mind in our industry is, of course, the tariffs, the duty, the 301 tariffs, which were started under the Trump administration and continued under Biden. It's kind of been a progressive tax on imports from China that started off small and a little bit negligible and have scaled up. And so, you know, every business, every importer is and should be concerned with their bottom line. And, you know, these punitive tariffs that were put in place by the Customs and Border Protection, they wanted to, they're enacted to encourage American businesses to divest from China and seek alternatives. So, you know, that's probably the main driver. But there are some other points as well.
Casey: Sure. And Alex, how have you seen the tariffs impact your business?
Alex: For me as a manufacturer, I would say it's a positive impact for me. We have many customers originally ordering from China due to the tariffs, the cost constraints and they are slowly starting to order from us, a non-Chinese source. So, like for business wise, it's actually a good thing for me. Yeah.
Ben: And yeah, I mean, I would just say, you know, the cost is one thing and that's, you know, first and foremost on everyone's mind. But there's other factors as well. And large companies, like Apple and Sony and multinationals all over the world that, you know, saw this and in kind of earnest and started divesting from China to alternative markets like here in Thailand or Vietnam, you know, more than a decade ago. But now it's kind of small and medium enterprises are making the switch and experiencing, you know, some growing pains associated with that. But, you know, ultimately, it's important for importers to be able to have free access to their goods, and things like increasing authoritarianism in China, clamp down on Western businesses, and just general saber rattling on the geopolitical stage all present pretty large risks to importers who are solely reliant on Chinese manufacturers for their imports.
Casey: I think you bring up a really good point because, you know, like you said, like 10, 15 years ago, these large companies like Panasonic and Samsung, I mean, they started moving their manufacturing a long time ago even before the tariffs, because, you know, I think they saw something coming. And it's true, like a lot of these SME companies, they're now making the jump and they're experiencing a lot of pains, you know, moving their supply chains. So I mean, with your business, is your factory capacity filling up quite quickly?
Alex: Yeah, we actually especially before Covid but especially after Covid, I think people seeing the situation in China. We constantly get more orders each year from like from compared to last year. We also our sales volume increased mostly from the redirected orders.
Casey: So, you know, I would like to talk about maybe the differences between the three countries that we actually work in. So we work in India, Thailand, and Vietnam. And I think, you know, every country has its competitive advantages and its noncompetitive advantages. So, maybe let's talk about the differences between these countries. Let's start off, Ben, like what do you see out of India?
Ben: Yeah, India, you know, it's a huge manufacturing market. It's probably the number two for heavy industrial outside of China. So, India is relatively new as an import source for American companies, but they've been exporting to countries in Asia, particularly in the Middle East as well as Africa, for a long time as the primary supplier. You know, India has a lot of foundries, a lot of forges, there's multiple regions that are kind of manufacturing hubs. At a really high level, you can get great stuff out of India, and it's probably the most cost competitive option of the three. Where you really have to tread carefully is choosing the correct partner. And, there's a lot of incorrect partners out there where, you know, they may promise you that everything is going to be great and you might get your sample, and it just doesn't always work out that way. And so, you know, a lot of what we do behind the scenes when we're not in a studio like this is we're in country. I've been to India four times this year. And we're driving around and we're visiting foundries. We're checking up on our existing projects and always looking for, you know, the next great supplier. And so, to try to just choose someone off of Google, it's a risky proposition. I would say you don't always have the best bet.
Casey: I would say in India, everyone says, yes, it can be done. And then after you sort of start digging down, you find out very quickly that they just say yes to everyone. Yeah. I think that's one of the biggest pitfalls that we see in India and also the infrastructure. You have to be very careful about picking a partner, not just for them, but also their location. You know, I'll give you just a quick example. We were auditing. We were going to an investment cast factory. And when we were going up the road, I noticed that it was dirt and also a lot of potholes. And India has a very strong monsoon season. So we were talking, we were like, well, how is a container going to get up this hill when it's the monsoon season? Because it's going to be muddy. So even like simple things like that, it's very important to know the factory, the layout and also the surroundings of the factory. So yeah.
Ben: Yeah. I think, you know, it's certainly helpful to have an advocate who has boots on the ground and does the hard part of weeding out the duds for you so that you can have confidence in placing your project in India and take advantage of all the, you know, advantages they have for low cost and high quality that certainly does exist.
Casey: What about Thailand?
Ben: Yeah. I mean, I think I'll pass this one over to Alex in a second because of course he has a factory here. Our experience in Thailand is that generally the communication is quite good. The level of English is certainly good, and the quality tends to be right at expectation. So, you know, it's more of a market where people do what they say and say what they do.
Casey: Good infrastructure.
Ben: Good infrastructure too. You know, some of the challenges are just benchmarking against those kind of target costs can be a bit of a challenge with, you know, a relatively higher GDP than surrounding markets. But, you know, it's a good place. I mean, Alex? Right.
Alex: For me, yeah, it's...our factory in Thailand, we rarely see power outages. So the infrastructure is pretty decent. And around the surrounding area, the roads, logistics is very convenient. You can have third party logistics and DHL like or just some like large FCO transporters like everything is in place, but there are some issues, for example, in some RFQ, right? Some parts might not just be casting like for casting, we can just use our like molding machines, and everything is in-house, but sometimes they are like forging parts or like a rubber parts. And then I have to go find suppliers in Thailand, right? And sometimes they kind of reply quite slowly, sometimes. Yeah. Or they don't really have to follow up from phone calls and maybe take some time. Eventually they will quote yes. But it takes some time in the requesting for the RFQ stage. Yeah. And the pricing sometimes is, sometimes our price, our casting parts, machining parts is in targets. It's right for right under the target price. Right. But the accessories, like the parts, stamping parts and a lot of different smaller parts that in the assembly with the casting. It's too high. Right. Yeah.
Casey: We've actually seen, it's kind of funny, like in India everyone says yes, but in Thailand it's very hard to get people to a lot of times to take a project because when they decide to quote and take a project, they do a very good job. But a lot of the times getting them to quote, they're very hesitant, a lot of Thai factories. Once they do it, they'll do it very well. But getting them to do it is sometimes tricky. Yeah. And I guess the third country. What about Vietnam? What do you see? Yeah.
Ben: Vietnam is definitely emerging. A lot of, you know, multinational companies are setting up factories there for both finished goods and industrial parts. In general, it kind of, I would characterize it as kind of being in the middle of the two countries we just described in terms of, you know, generally being a little cheaper than Thailand and generally being a little bit more transparent and communicative than India. Like we touched on earlier, you know, there's a little bit different offerings in terms of the manufacturing processes there. There's fewer heavy industry options like forging or casting. It's more injection molding or machining generally. There are some some larger old forges in the north that, you know, the other challenge with Vietnam is it is kind of still, you know, it's geographically it's a little bit of a challenge, because in Thailand you use kind of one central port no matter where anything is being manufactured. But in Vietnam, there's a main port in the north and a main port in the south, and so that can make things like consolidation tricky. It can also make things like subcontracting, you know, if your coating supplier is in the south, but your foundry's in the north, it's a lot of roadways to cover. And the infrastructure is not amazing for facilitating that yet. There's some changes in the works. High speed rail from the north to south of Vietnam, we're looking forward to. But yeah, it's a geographical challenge.
Casey: I think, we've talked to a lot of customers in the US and when they fail in Vietnam, it's a lot of the times, because they don't have a team there, because the English level in Vietnam is not so good. So it's really critical to have a good team in place there and a local team, a local Vietnamese team there to manage the factories. So yeah, we're we're lucky to have a good team in Vietnam to help us with that. Alex is actually one of our manufacturing partners, and we've worked with Alex on a few different projects. Ben, why don't you tell them about one of the projects we did and how we helped consolidate and save our customers some money? Yeah.
Ben: I mean, I think, it's the most recent project we did where we were producing parts that are ultimately used in machinery, and that machinery is specific for the braided hose industry. So we're supplying parts to a manufacturer in the United States who assembles those parts and turns them into a product that's then used all over the world. It's quite a precise product with a lot of challenging tolerances and engineering requirements. We worked with Alex and his company, Maesot Heavy to produce the parts and, you know, that's super helpful. Being that we're all locally based here, it's very easy for us to go on site to communicate in our own time zone, and to, you know, go up and inspect the goods, make sure that everything is according to the print and drawing requirements, and ultimately, we were able to help this particular client by consolidating with another foundry that they work with, with a different style investment casting foundry. And we were able to consolidate and save a lot of shipping costs. Shipping costs are are always kind of fluctuating, rising and falling. But, if you are able to consolidate it, you can create more frequent shipments that are more efficient. They just have take up. You can use the cubic meter allotment a lot better. So we were able to do that. And, that was extremely helpful for our customer because of the nature of their business, they produce a large mix of products at quite low volumes. And so it's important for them to support their just-in-time inventory to work with MOQs that make sense, and we were able to offer savings on logistics by consolidating with another process of casting.
Casey: What immediate actions should companies consider to avoid potential pitfalls in their relocation efforts?
Ben: Yeah, I would say, you know, choosing the right partner is critical. We have countless examples from our clients, horror stories where they've chosen the wrong partner, and, you know, depending on where you're going, it could be anything from like, you know, most of them are pretty good to it's a total crapshoot. And 99% of them are pretty bad. I think there's, Casey you and I have stories of being in India, to meet a prospective vendor that we felt really good about, and had been chatting with online for weeks. And, you know, we pull up to the shop and we know from the second we arrived that it's just not going to be a fit. And we, you know, we just tell our driver to put it in reverse and head on to the next meeting because, you know, it's tough to know who the right partner is. That's why we feel it's important to have the local presence. Go ahead.
Casey: Well, yeah, I was going to say, I think the reason Alex is a really good partner and we've seen this especially in Thailand. Some of these factories, they've been around for decades and some of the children are running the factories now. You know, their dad's given the reins and the children are not really hungry for business. So, Alex is great because, you know, you're very hands on. You know, you're at site, and, you know, when you're partnering with someone, it's really important to to partner with a factory owner that, you know, is hands on and and knows everything about, you know, their operation. Yeah.
Ben: That that ties straight into like, you know, we always talk about in the office about the three Ps. And, you know, the Ps are, they're 'Process', which is, you know, all of your manufacturing infrastructure, your documentation, your quality programing, which is super hyper critical. Then there's 'Price', which is important to every importer, and then but the last one is the 'People', and you need to have people who are flexible, who are willing to solve problems, to dig deep and investigate. Maybe it's not something they've done before, but, willing to study it and, you know, run a feasibility study, see if it's going to be possible, try to find potential solutions to be able to turn these concepts into cast goods or forgings or anything like that. And I think, the three Ps, the 'People' is really important. All three of those are very important. But the people is one you definitely can't miss. Yeah.
Casey: And like going into like, you know people it's hyper critical like especially you know, communication is of the utmost, you know, importance and you know, just being able to, you know, just work always on WhatsApp with, you know, all of our partners, you know, seeing what's going on, you know, send us a picture or we have to go, you know. So yeah, having a good personnel at the factory is very important. Like Alex, when you're working with companies like ours. What do you see as important to partner with a company such as ours? Right.
Alex: For us, the number one quality that we hope that we can get from our customer or partner would be like be very clear on the specification from the start. Because, like from day one, it would be the RFQ. Right. Very first step, RFQ.
Casey: And what needs to go into the RFQ to make it clear? Right.
Alex: So in the RFQ we need the drawing, the material, the manufacturing process. What kind of QC standards are we talking at. Because that will all affect the price. And some customers, they are either unsure or they don't have the right person on this as the coordinator. So it's kind of frustrating for us. Like, okay, so what kind of quality are we talking about? What kind of process are we talking about? Because we have to write the code with our company name on it at the end of the day. So it's really great working with Align Manufacturing too, like from the emails and the information and the communication is very clear that we know from there what we are working with. If Align Manufacturing, if you guys did not get the information from the customer, we immediately get the feedback. Usually Align will contact the customer and get us that feedback and have been really, really helpful.
Casey: Yeah, I think like, what we found is like, you know, working with, you know, factories and guys like you is like it evolves into more of a like we're on the same team. You know, we all have the same goal. So it's important having that clarity. Yeah, so I mean you know we always try to stress to the OEMs or customers in America, don't wait until November for the election. Like, let's like you should start now. You know, like, let's get going. Why is that these OEMs need to move quickly?
Ben: Yeah. I mean, I can understand why people would want to wait and see. This election it involves importers more drastically than probably any other election in history that I can think of. And so there's a tendency to want to wait, but I think that's a counterintuitive tendency. And that's for a few reasons. I think the biggest reason is that there's only a fixed number of factories. And when you look at that cross-section of factories and talk about which ones are actually good, it's a very, very small percentage and capacity at those factories is filling up. And they're filling up from all the first movers and everybody who already moved. And so, you know, importers who are making the switch now, they're frankly, a little bit late to the game. It's not too late, but the longer you wait, kind of the worse your options will be. And you don't want to be stuck with the wrong manufacturing partner. And, you know, on the flip side, the same point of that is, you know, pricing follows, pricing is the same anywhere, it follows scarcity. And so as capacity decreases, price increases. And you better believe if you're bidding, you know, if Trump wins and a 60% tariff is enacted, your factories are going to know that. And when they're pricing out your quotes, they're not going to be pricing out according to benchmarking your 2017 China price. They're gonna go ahead and tack on an extra 60% to that knowing that they're still competitive. Ad so, you know, moving now is very advantageous for importers to get the correct manufacturer and the correct price. And for those who, you know, want to wait and see, that's a game that any, any business is allowed to play. But it's kind of a 50-50 at this point. And, it's like going to Vegas and betting on red. It might be fun for a weekend, but it's probably not something you should do with the future of your business, especially if it's a product focused business.
Casey: And I think, especially like you're looking at timelines, you know, I mean, how long does tooling take usually at your shop, right.
Alex: Um, for the complicated one, it depends on what you do. We we are foundry. Right. So we have the pattern and then the if it's a complex with a cavity and or a different like undercut geometry, we have to have the core box. Right. So if it's just one model maybe, maybe right now we're kind of busy. So maybe around two months for the medium to simple one. Right. But if it's really complex with like multiple multiple core box, many cavities then maybe it's maybe, like, maybe three months. Yeah.
Casey: And that's the thing. I mean, that's that's just the tooling. And then we have to make the sample and then the customer has to approve the sample. And, you know, sometimes the T-Bone sampling doesn't always get approved. So you're just moving the timeline. And so it's yeah, it's really important.
Ben: And there's third party testing and a million variables that can push that timeline out. And so, you know, even if you were to start today, you're looking at, you know, first production shipping out around the election anyway. So um, it's a, you know, it's just kind of, uh, urgency and expediency is is advantageous in this situation.
Casey: We talked about the 301 tariffs, but like what are some other reasons why manufacturers need or should be moving out of China?
Ben: Yeah. I mean, we kind of touched on this. But you know, when we talk about these other factors besides the tariffs, these are what we're driving the large corporations out of China in the first place, before the trade war and before the pandemic. And there's a lot of them. There's a lot of macro trends that are leaning away. There's increasing cost of labor in China as the economy develops there. The cost of labor is is increasing. And of course, that affects the bottom line in these very labor heavy industries. There's an aging workforce associated with the demographic changes in China. And so that's going to reduce the availability of factory workers who are interested to work in the manufacturing industry. And then there's, you know, kind of the red hot one that nobody really wants to talk about. But it's, you know, in the event that kind of what this kind of cold tit for tat trade war that's going on, if that escalates into something hotter, you know, where there's a real conflict, whether it be, something with the US or something with Taiwan, something in Hong Kong or all of the disputed South China Sea territories. You know, all of these are kind of red hot button issues. And if a conflict goes from cold to hot, you can pretty much kiss your supply chain goodbye. And it doesn't matter if you're willing to pay the tariffs at 30% or 60% or 100%, you just won't be able to get it. It'll be like the beginning of the pandemic, but it lasts forever.
Casey: I think it's important for us to say as well, like we're, these are all like facts. We're not against China at all. I mean, I used to live in China for a few years. I really like the Chinese people. But I think the point of this conversation is to really highlight the need to get out, at the very least.
Ben: Yeah. And I think it's, you know, something that's often overlooked or just not always talked about is that the US has placed China as a designated US foreign adversary, and that's a very short list. There's only seven countries on that list. It's North Korea, it's Iran, it's Cuba, it's Venezuela. And China is on that list. And when you look at the trade between the United States and these other countries that have been designated as an adversary, it's virtually nothing. We have strict embargoes against all of these countries. We're not allowed to import anything. It's not a matter of duty. It's just you can't get it. And the fact that the US government has placed China on this list, and most recently was said that it's actually the most potentially dangerous foreign adversary on the list.
Casey: But yet it's our biggest trade partner.
Ben: Our largest trading partner kind of knocked some reasoning behind this policy, which not everyone kind of understands the why or the how, but, you know, that's kind of the why. And the US government is interested in making sure that they can pursue their foreign policy interests without affecting US businesses. And, you know, US businesses would benefit from falling in line with that. The US government is going to keep these in place as long as China is on that list. And yeah, the government wants you to divest.
Casey: The last sort of question I would have for you guys is, you know, how do you see, like the landscape of global manufacturing evolving, you know, as this relationship between China and the US is decoupling?
Ben: Yeah. I mean, I would say, a lot of the growing pains that importers are having now come from working with less developed, less established factories that are not as familiar with the documentation requirements or the expectations and standards of the importers. You've got to remember that when China first started out, people didn't have very nice things to say about Chinese quality or Chinese suppliers. And that changed. And I expect the same thing to happen in our markets. As you know, these factories become more accustomed to working with Western importers. And, I think that some of those growing pains will change. I think we'll see new types of factories emerge as well, where right now we have mostly process-based factories that, you know, it might be a foundry, it might be a forge, it might be a machine shop. But those generally create parts and not products. And when you're talking about a packaged consumer good or even a complex assembly of an industrial good, that's a different kind of factory. That's an assembly factory. And we are seeing those emerge especially for joint ventures that are, you know, getting set up across the region. But I think you'll also see that as an independent job shops for contract manufacturing.
Casey: Yeah, that is a good point. I think, you know, a lot of the times you go to, you know, you go to a Costco or a Target and you, you see, you know, just as you think it might be so simple, but assembly is just it's extremely complex. And setting up an assembly in a factory, it takes time for it to evolve and be mature. So, yeah. Well, do you guys have anything else that you'd like to add into the conversation?
Ben: I, yeah. I mean, just on your last point, you know, other changes, I think you could see, some more friendly trade policies. You know, India has really been the shining star of this, to really recognize this opportunity and seize it with really manufacturing forward policies. The Made in India policy, which grants, you know, interest free loans as well as all kinds of tax incentives for manufacturers. I think we'll see that deployed across the region and in India. We'll see them continue. Right. Ease of doing business. I see it improving where there may be, you know, importation of child parts to be assembled or the allowance of purchasing a child part domestically. But having it be VAT exempt because it's ultimately being exported. So I think we'll see some change in the regulatory environment in these countries, which are catching on to the opportunity that's there. And it's just it really is a massive opportunity.
Casey: Yeah. I really appreciate you guys joining me. It's been a really nice conversation. Alex came all the way down from north Thailand to join us on this podcast. So Alex, thank you very much for joining us. Thank you for joining us today. If you found this discussion insightful and are considering how best to navigate the complex manufacturing shifts, I encourage you to read our recently published report Guiding US-Based Manufacturers Out of China. It's packed with in-depth analysis, real-world case studies, and practical strategies to help you make your informed decisions. To discuss your specific needs, don't hesitate to get in touch with us at Align Manufacturing. Together, we can ensure that your transition is smooth and successful. Thank you again for watching and we look forward to helping you secure your manufacturing future beyond China.
For a more in-depth exploration of these themes, download this Align Manufacturing report:
Innovations Transforming Investment Casting
Investment casting, also known as lost-wax casting, has existed for centuries in manufacturing. This is a process for casting metallic parts with great precision and detail; it is, however, a somewhat old format compared to newer versions. This article takes a look at some of the recent developments in investment casting today, with a special focus on 3D printing and new material technologies.
Lost-wax casting is a manufacturing process that produces a wide variety of products of complex geometries. It generally involves a wax pattern surrounded by a ceramic shell. When the shell hardens, the wax is melted out to leave a mold. Hot metal is poured into the mold and the casting can be completed. It is highly commended for products that have excellent surface finish quality and have close tolerances. It is applied in several industries, from aerospace and automotive to medical, where precision, quality control and automation take the foremost place.
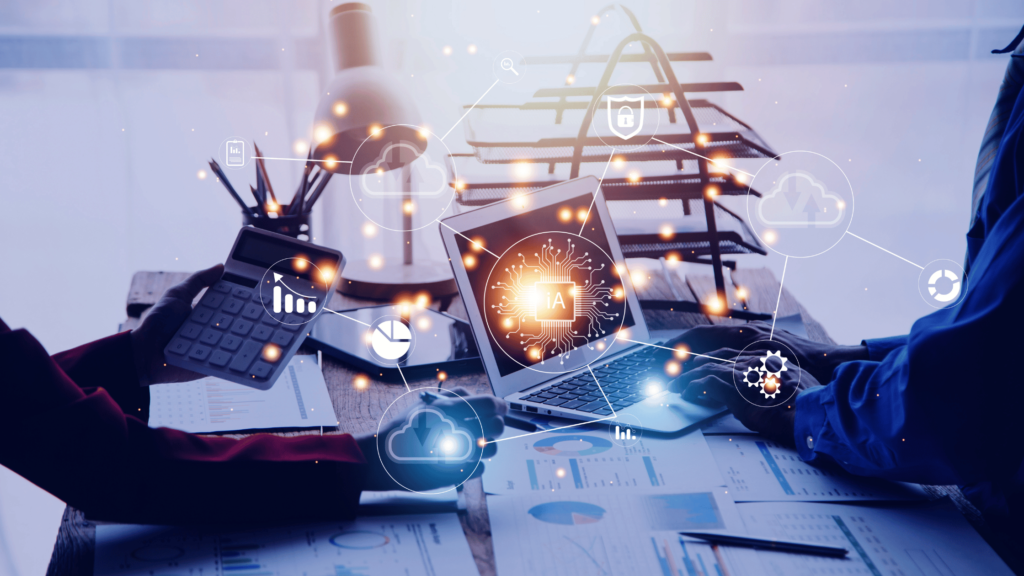
Historical Context and Traditional Methods
Historical origins date back over 5,000 years to ancient civilizations like Mesopotamia and Egypt. Traditional investment casting usually involves several labor-intensive steps:
- Pattern Creation: A wax pattern has to be made that is a replica of the final part.
- Assembly: Several wax patterns can be attached to a tree-like configuration.
- Shell Building: The wax pattern is to be dipped into a ceramic slurry and coated with sand to produce a hard shell.
- Wax Removal: The shell is heated to melt and drain out the wax to leave behind a hollow ceramic mold.
- Casting: Molten metal is poured into the mold.
- Finishing: The ceramic shell is broken away from the cooled metal and the metal part is finished.
While effective, this traditional method is time-consuming and requires significant manual labor. Innovations in technology are addressing these challenges and transforming the process.
3D Printing: A Game Changer in Investment Casting
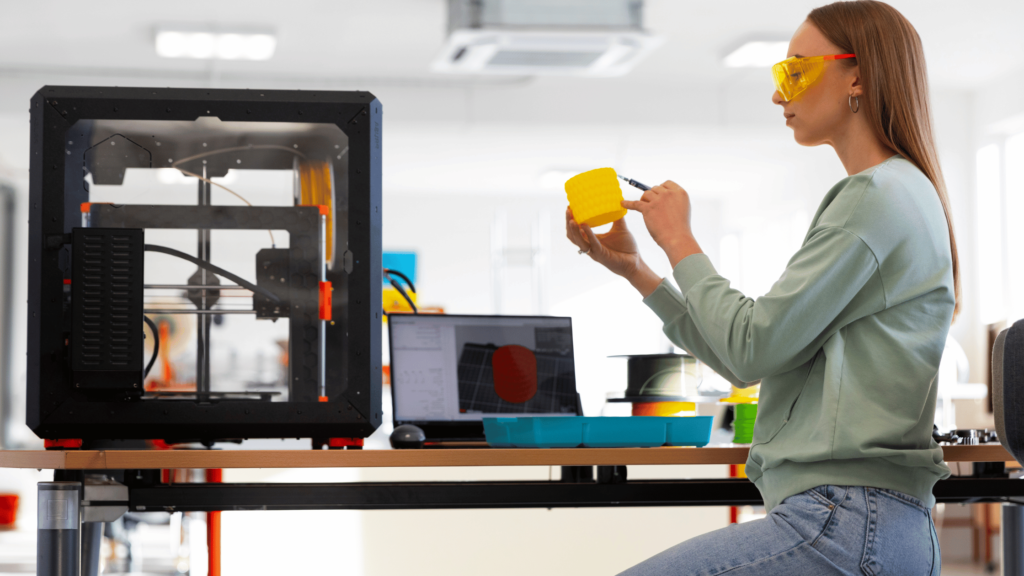
Application of 3D printing or additive manufacturing in the investment casting has significantly revolutionized the process. Earlier, the wax patterns were made by molds and were laborious and, on occasion, costly, especially for something with complex shapes. 3D printing avails a cost-effective and quick alternative.
Advantages of 3D Printing in Investment Casting
- Design Flexibility: It is perfect for creating complex geometries, impossible or very hard to create using traditional methods.
- Reduced Lead Time: The application of 3D printing to make patterns nullifies the need to make the molds, and this way, it reduces the time taken to manufacture patterns significantly.
- Cost Effective: In case of low volume production itself, or prototyping, 3D printing can turn out to be more cost-effective than the traditional lost-wax method in cases of tooling.
- Parts Customization: it has easy parts customization. No additional tooling, neither molds are needed.
3D Printing Technologies in Use
There are several 3-D printing technologies applied to investment casting, each with its unique sets of benefits:
- Stereolithography (SLA): This makes use of a laser to cure liquid resin into solid shapes. It can come up with never seen before detailed and accurate patterns.
- Selective Laser Sintering (SLS): It is a laser kerosene that fuses powdered material, layer by layer, to make powerful and robust patterns.
- Fused Deposition Modeling (FDM): In the FDM process, the melted material gets extruded and laid down to construct the layers. The cost-effective and rapid method to build patterns which are relatively simple.
New Material Technologies
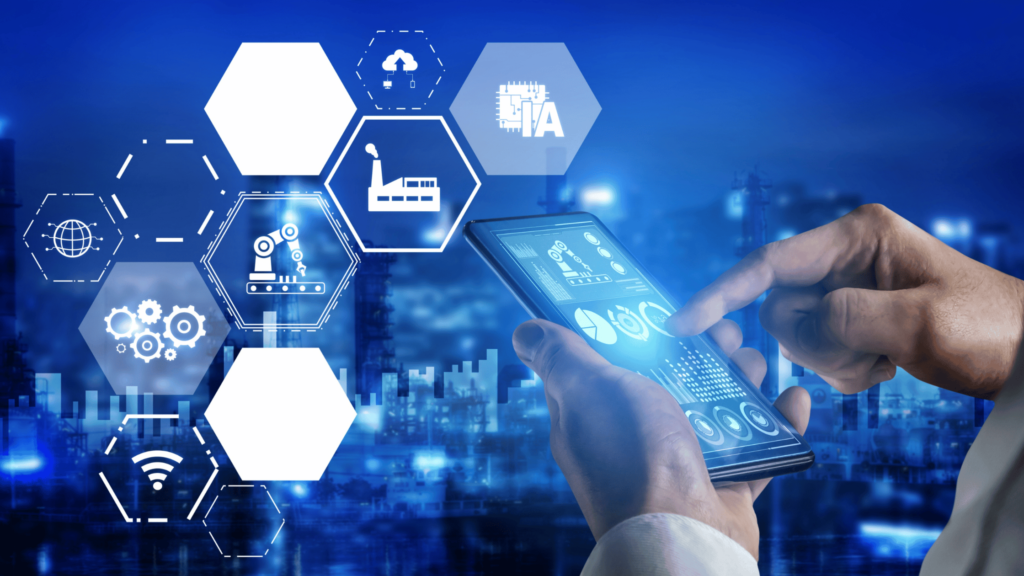
Advancements in material science are also revolutionizing investment casting. New material and new alloys are making the cast parts of better quality, robust, light, and durable.
High-Performance Alloys
New high-performance metals are manufactured to cater to the demanding needs of industries ranging from aerospace, automotive, and medical. These new materials boast improved mechanical properties, corrosion resistance, and temperature tolerance.
Ceramic Shell Improvements
Advanced ceramic shells revolutionize the investment casting process. Improved ceramic formulations enhance the strength and stability, thus reducing the risk of defects and increasing the quality of the cast parts.
Benefits of Advanced Ceramic Shells
- Higher Strength: Improved ceramic materials can withstand higher temperatures and mechanical stresses, allowing for the casting of more robust parts.
- Reduced Defects: Enhanced formulations reduce the occurrence of common casting defects such as cracks and inclusions, leading to higher quality parts.
- Faster Production: Advanced ceramics can shorten the shell-building process, increasing production speed and efficiency.
Integration of Smart Technologies
The integration of smart technologies, such as the Internet of Things (IoT) and Artificial Intelligence (AI), is also making its way into investment casting.
IoT and AI in Investment Casting
- Real-Time Monitoring: The Internet of Things will monitor the casting process in real time by means of different built-in sensors to provide data for maximizing production and minimizing defects.
- Predictive Maintenance: Using AI algorithms to analyze, equipment breakdowns could be predicted, and timeously fixed before they occurred. Reduces downtime and maintenance costs.
- Process Optimization: AI can analyze production data and detect inefficiencies. It can even suggest alternatives, enhancing the general efficiency of the process used in casting.
Future Trends
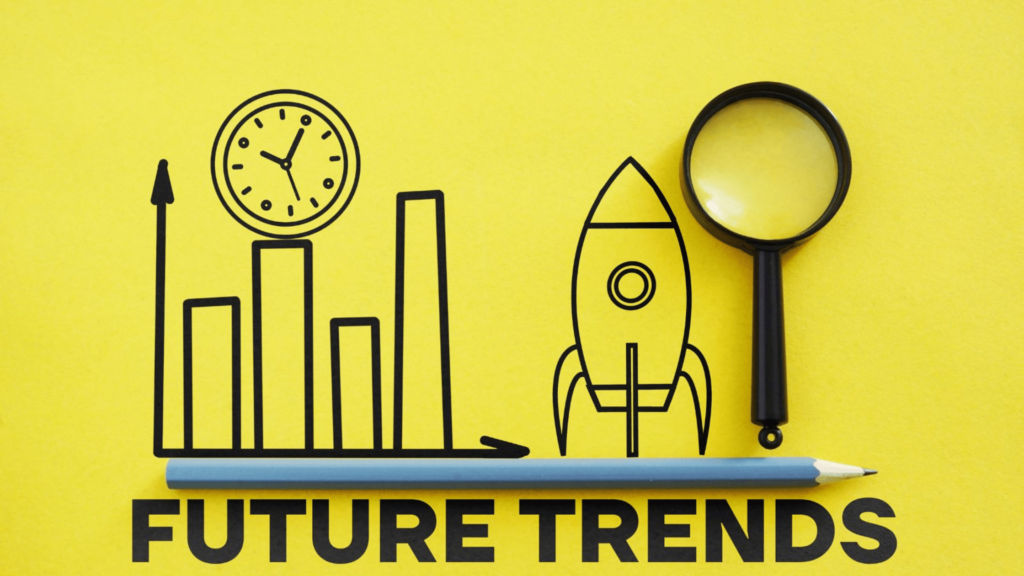
The future of investment casting looks promising, with continued advancements in 3D printing, material technologies, and smart manufacturing. As these technologies evolve, they will further enhance the capabilities and applications of investment casting, opening up new possibilities in various industries.
Emerging Technologies
- Digital Twin Technology: This is the technique for the development of a digital replication or model of physical process operation. This helps to monitor internal operations in real time for optimization. In general, it can help in the prediction and prevention of potential problems that would occur.
- Sustainable Practices: Developments of materials and processes are also done in sustainability. A conductive approach towards eco-friendly materials and the recycling of the materials used in the casting is reducing the impact of investment casting on the environment.
- Hybrid Manufacturing: Traditional techniques used in casting are combined with modern-day techniques, like 3D printing, to design hybrid manufacturing processes. This develops efficient production and better-quality parts.
Conclusion
Investment casting is indeed being transformed through innovative technologies. Other applications of 3D printing and new material technologies besides improving efficiencies and qualities within the manufacturing process continue to expand the applicability of the casting process. These innovations will undeniably be the logos of the manufacturing future that will thus make the investment casting process more flexible and effective than ever before.
Automation and Quality Control in Investment Casting
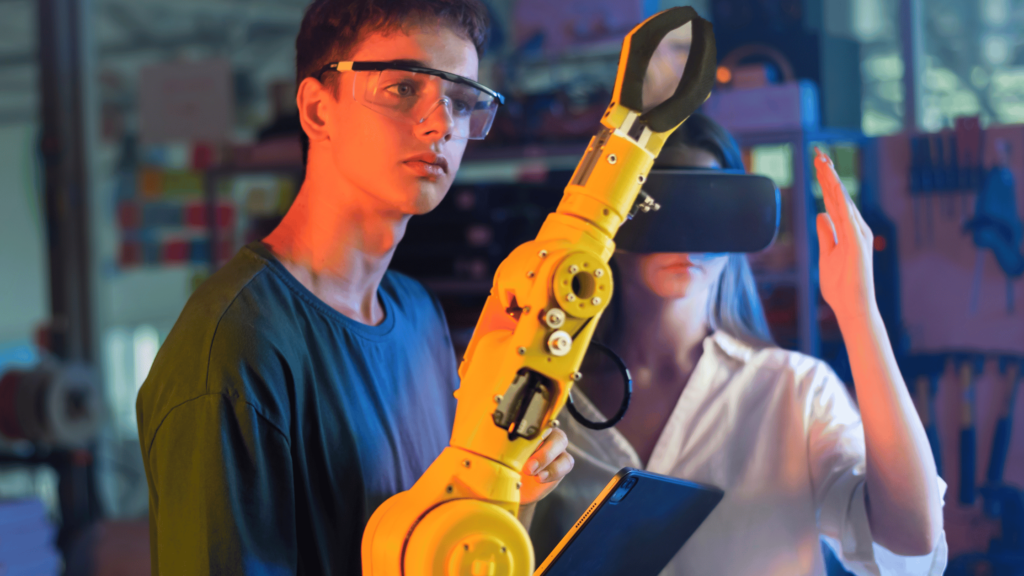
Investment casting, also known as lost-wax or precision casting, is one of the oldest doctrines to intricately craft components. Though the origin of the process can fold many centuries back, it does not necessarily mean that it is antiquated thereby. The improvements and changes made have been brought on by automation and advanced quality control. These have improved the areas of efficiency, precision, and part reliability, all of which contribute to the competitiveness of investment casting in this high-paced manufacturing environment. This blog goes through the aspects of automation: its role in the enhancement of the process and the critical quality control measures that ensure part reliability.
The process of Investment Casting
Investment casting is a manufacturing process for generating complicated and high-precision components. Such a process starts with the creation of a wax pattern, which is an exact replica of the desired part. The pattern is then coated with a ceramic shell to fashion a mold. After this ceramic shell hardens, wax is melted out of it, leaving a cavity that is then filled with molten metal. Later on, after it solidifies, it is to be broken away to reveal the final casting. The process is marked by its ability to generate parts with high surface finish and tight tolerances, which qualifies it to be applied in critical industries such as oil, railway, construction, and industrial sectors.
The Role of Automation
Automation has revolutionized many aspects of the lost-wax casting process. Manufacturers no longer have to rely on the human hand for repetitive and precise tasks. Instead, automated systems have been incorporated, which allow them to achieve higher levels of precision while reducing lead times and improving overall efficiency. Outlined below are some of the ways in which automation is bringing much improvement to precision casting.
Automated Pattern Creation
Historically, the making of wax patterns has been a very labor-intensive process. However, this process has been improved with automation to a great extent. Automated pattern making machines can produce wax patterns with remarkable tolerance and repeatability based on computer-aided design data.
- Faster Delivery and Shorter Production Time: Automation processes the pattern making at a quicker rate, thus reducing the lead time for production.
- Makes Patterns of Complex Geometries: Machines are capable of producing complex geometries for the patterns of designs that would not either be possible or practical to reproduce using manual techniques.
Robotic Shell Building
Another area of the process that has been revolutionized with automation is the shell building process in making the ceramic shell around the wax patterns. The robotic system carries out the wax pattern’s dipping, draining, and stuccoing (coating with ceramic).
- Higher Precision: Automation ensures precise control over the amount and parameters of pouring. Since molten metal is poured into ceramic shells, automation can maintain the right temperature of molten metal thus preventing defects due to poor pour temperature.
- Even Pouring: Delivering molten metal through robots at a steady pace prevents defects like entrapment of air or incomplete fills.
- Safety: Since automation prevents accidents due to molten metal handling, the workers’ safety is ensured.
Quality Control Measures in Investment Casting
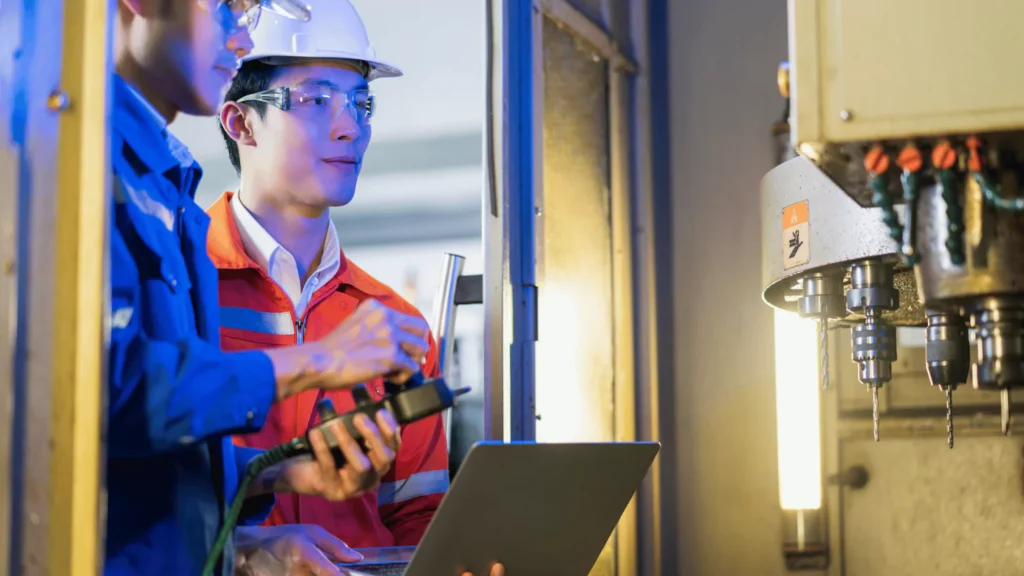
Quality control involves various critical steps, from inspection of patterns in the initial stages to the final evaluation of the products. In a nutshell, the following is major quality control measures in lost-wax casting:
Pattern Inspection
The quality of the final cast part depends on the tolerance of the wax pattern. Inspection of the wax pattern is hence an important step.
- Dimensional Criteria: The patterns are gauged for dimensional features, and modern gauging appliances like CMM are used for inspection.
- Surface Condition: Scrutinizing the surface of the wax pattern for imperfections prevents flaws from appearing in the final casting.
Shell Integrity Testing
This is critical in the case of the ceramic shell used in the casting process. Any defect in the shell may result in defects in the final part.
- Visual Inspection: The shell is visually inspected for cracks, incomplete covering, or some other defects.
- Non-Destructive Testing (NDT): Ultrasonic testing and radiographic inspection help to detect internal defects in the ceramic shell.
Metal Quality Control
The quality of the molten metal is another important factor in casting. Quality metal is free of impurities and has the right composition to produce quality cast parts.
- Spectroscopy: This will be applied in analyzing the chemical composition of the metal to assure that it meets the required specifications.
- Inclusion Analysis: The analysis is carried out using such techniques as optical and scanning electron microscopy to detect non-metallic inclusions present in the metal.
Post-Casting Inspection
After the metal has been poured and the ceramic shell has been removed, these post-cast inspections help ensure the quality of the end product.
- Dimensional Testing: Checking the dimensions of the cast part to verify with the specifications undertaken.
- Surface Testing: Testing the cast part for cracks, porosity, or surface roughness.
- Mechanical tests: Test and confirm that mechanical properties, including, for example, tensile strength and hardness, are as per or meet the specified in the required specification.
Advanced Automation Techniques
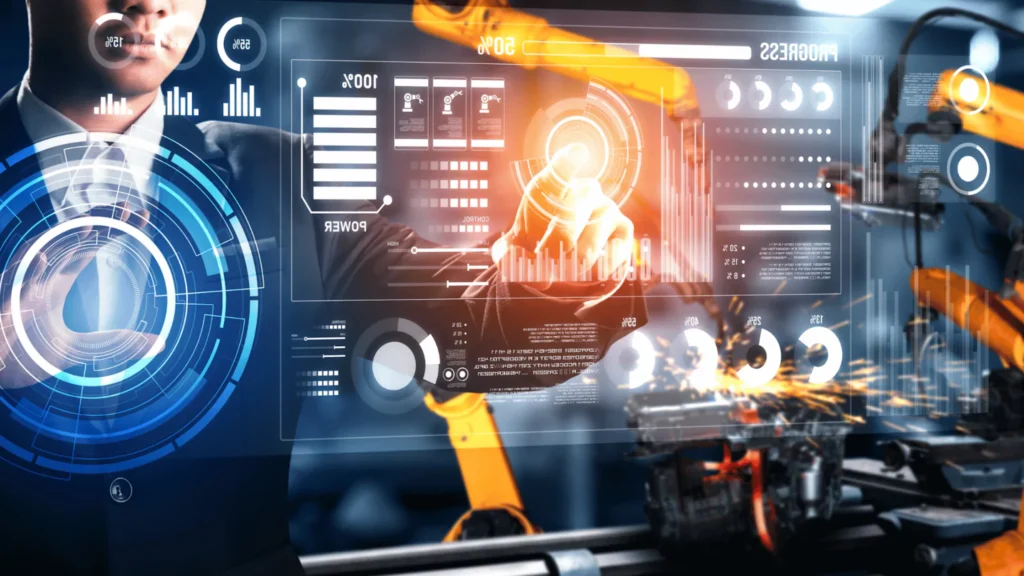
The adoption of advanced automation further elevates the process. Artificial intelligence, machine learning, and the Internet of Things are some commonly used concepts.
Artificial Intelligence and Machine Learning
In the context of casting, artificial intelligence and machine learning can be harnessed to study the huge data that comes out from the casting process to note the patterns and sometimes even predict results. The manufacturers employ it for improvement in process and quality control.
- Predictive Maintenance: Predicts when equipment is likely to fail, allowing maintenance to be planned in advance, thereby reducing unplanned downtime.
- Process optimization: ML algorithms can analyze process parameters and suggest adjustments which enhance yield and reduce defects.
- Defect Prediction: AI can predict the likelihood of defects in cast parts and allow early intervention and corrective measures.
Internet of Things (IoT)
IoT is about implementing a setup to help collect data regarding various parameters around the casting process. IoT sensors monitor the temperature, pressure, and other critical parameters of the casting process in real-time. Data collected by IoT can further be analyzed for trends, detecting anomalies, and optimizing processes. This process can be adjusted and controlled remotely, allowing for interventions from a distant location.
Automation and Innovation in Critical Industries
Automation and quality control in investment casting have brought transformative changes, particularly in critical industries. These industries demand high precision, reliability, and innovation, and it has risen to the challenge.
Digital Twin Technology
Digital twin technology means a fully detailed virtual copy of the foundry process. The model is digital, allowing for process simulation and optimization in real-time.
- Process Simulation: The digital twins can simulate various situations, through which the manufacturers can optimize process parameters.
- Predictive Analytics: Data from the digital twin is subjected to analytics to allow manufacturers to make predictions concerning possible failures and, in that way, take preventive actions.
- Continuous Improvement: The foundry process can be continually monitored and improved through digital twin technology, thereby increasing process efficiency and quality.
Sustainable Manufacturing Practices
The current trend in the manufacturing industry is a move toward more sustainability. Automation and quality control make possible more sustainable lost -wax casting. An automated process ensures optimization in the consumption of energy and, in return, minimizes the environmental impact brought about by the casting process. Quality control measures will also help reduce defects and scrap, thus reducing waste materials and conserving resources. Material science has not been left behind in this quest, and more eco-friendly casting materials have been developed.
Conclusion
Automation together with quality control has created the most significant changes in the industry of investment casting. With the help of advanced technologies, the manufacturer can achieve the highest levels of precision, efficiency, and part reliability. Automation of casting steps, starting from pattern creation up to pouring, and quality control of the results of every step, streamlines the process, ensuring that every part meets strict standards. Financial investment casting will only shine more with the constant development of technologies such as AI, IoT, and digital twins. The innovations will put manufacturers well in the race in a market that is rapidly changing and in regard to top-quality components meeting high requirements, especially in the aerospace, automotive, and medical industries. It is expected that the merging of automation and quality control will bring further improvements in functionality and sustainability.
The Materials Behind Investment Casting
Investment casting is a widely used manufacturing process that allows the production of intricate and complex metal components with high precision. This process involves creating a wax pattern of the desired part, coating it with a ceramic shell mold, and then melting the wax out to create a hollow metal mold. The mold material is then filled with molten metal to produce the final component.
One of the critical aspects of lost-wax casting is the choice of materials, both for the wax pattern and the shell, as they directly influence the quality, integrity, and performance of the finished product. In this article, we will explore various precision casting materials, including plastic pattern materials, shell materials, and the metals commonly used for casting.
We will delve into their properties and characteristics, highlighting their suitability for different applications and discussing their advantages and limitations in the investment casting process.
Advantages of Investment Casting
Precision casting is a highly advantageous manufacturing process, known for its ability to produce precision components with intricate and complex shapes that other methods may struggle to achieve. One of the standout benefits of this technique is its exceptional dimensional accuracy and the ability to maintain tight tolerances, which are essential in industries such as aerospace and medical devices where precision is paramount.
Additionally, investment casting can utilize a vast range of materials, providing flexibility in application across various industries. The process also allows for excellent surface finish, reducing the need for additional machining and finishing steps, which can significantly lower production costs.
Moreover, the versatility in size and weight of the components that can be produced makes it suitable for both small and large parts, enhancing its applicability for different product requirements.
If you want to know more about investment casting, check out our article about The Basics of Investment Casting: An Introduction.
But for now, let’s dive into the different materials that can be used for the different casting methods.
Stainless Steel
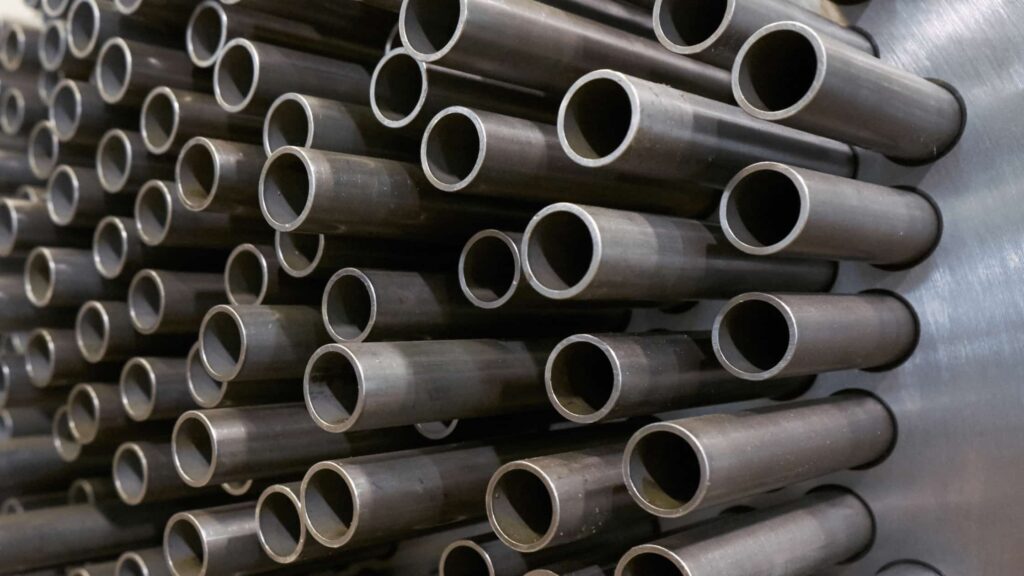
It is a versatile material with numerous applications and advantages. Its primary characteristic is its superior durability, making it ideal for various industries. One significant area where stainless steel is extensively used is investment casting.
In the automotive industry, stainless steel is utilized in gearbox parts and gears. The high strength and corrosion resistance of it ensure that these components can withstand the demanding conditions of a vehicle’s transmission system. Moreover, stainless steel’s resistance to heat and wear makes it an excellent choice for cam components, which are essential for controlling the opening and closing of valves in an engine. Golf club heads also benefit from stainless steel’s durability and resistance to corrosion, ensuring longevity and performance on the golf course.
Compared to other materials, it offers numerous advantages. Its corrosion resistance eliminates the need for maintenance and frequent replacements. Additionally, it can withstand high temperatures, making it suitable for applications where heat resistance is crucial. Furthermore, the aesthetic appeal, with its shiny and clean appearance, adds to its desirability in various industries. Overall, stainless steel’s unmatched durability, corrosion resistance, and versatility make it a highly sought-after material for investment casting process, automotive gearbox parts and gears, cam components, golf club heads, and many other applications.
Low Alloy Steel
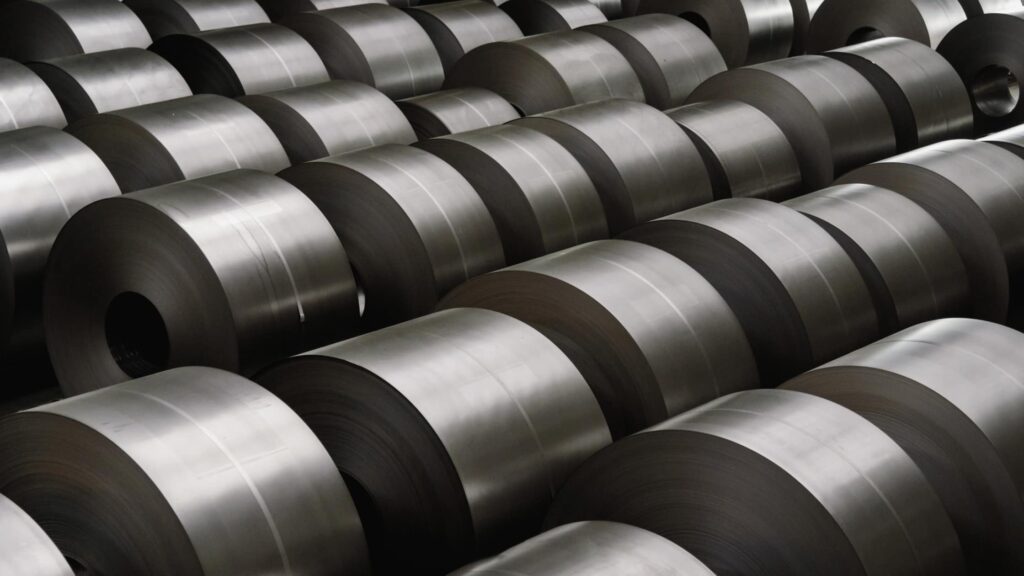
Low alloy steel, as the name suggests, is a type of steel that has a low percentage of alloying elements, usually less than 8%. This makes it more affordable compared to high alloy steels, which contain higher amounts of alloying elements.
It offers numerous benefits due to its beneficial mechanical properties. It has excellent strength and toughness, making it suitable for demanding applications where high strength and impact resistance are required. Additionally, it has good corrosion resistance, making it ideal for use in corrosive environments.
One of the key advantages is its suitability for specialized heat-treating processes. These processes involve altering the microstructure of the steel to enhance its properties. For engineered parts, low alloy steel can undergo specific heat treatments such as quenching and tempering to achieve desired hardness, strength, and wear resistance.
The applications are diverse. It is commonly used in the automotive and aerospace industries for parts that require high strength and durability, such as engine components and aircraft landing gear. Low alloy steel is also widely used in construction, oil and gas, and manufacturing industries for structural applications and machinery components.
Aluminum Alloy
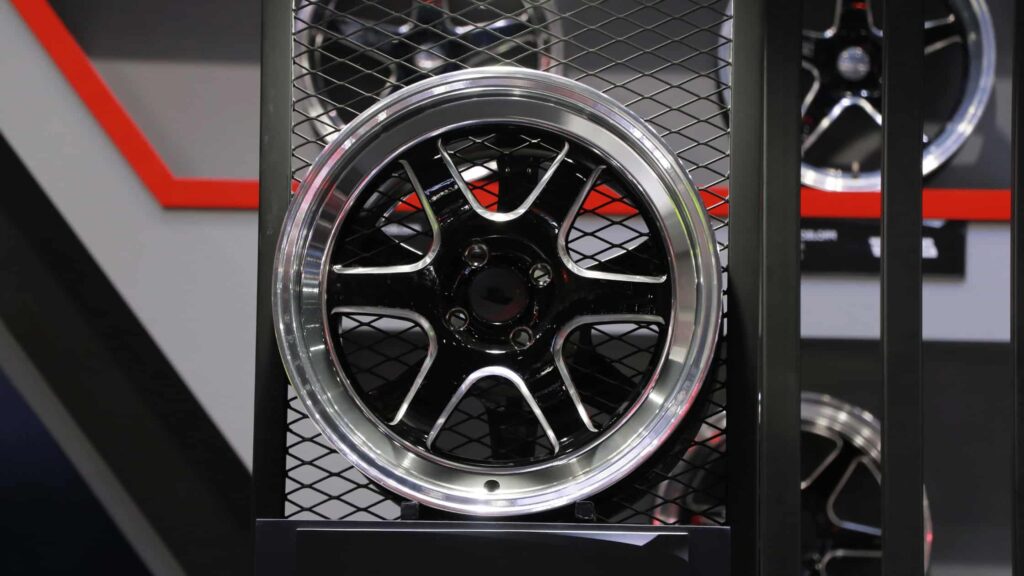
Aluminum alloy is a versatile material widely used for investment castings due to its beneficial properties and wide range of applications. Its key advantages include excellent strength-to-weight ratio, corrosion resistance, and high thermal and electrical conductivity.
Investment castings made of aluminum alloy find applications in various industries, including automotive, electronics, and industrial equipment. In the automotive sector, they are preferred for their lightweight nature, which helps improve fuel efficiency and reduce emissions. They are commonly used for components like engine blocks, cylinder heads, and wheels.
The blend of aluminum, silicon, and magnesium greatly enhances the strength and suitability of aluminum alloy for these applications. The addition of silicon provides improved fluidity during the casting process, making it easier to achieve intricate and complex shapes. Magnesium, on the other hand, increases the alloy’s strength and ductility, allowing it to withstand high-stress applications.
In electronics, aluminum alloy investment castings are used for heat sinks, connectors, and housings, as they possess excellent thermal conductivity, ensuring efficient heat dissipation and component protection. In the industrial equipment sector, these castings are employed in pumps, valves, and machinery components, benefiting from the alloy’s durability and resistance to wear and tear.
Carbon Steel
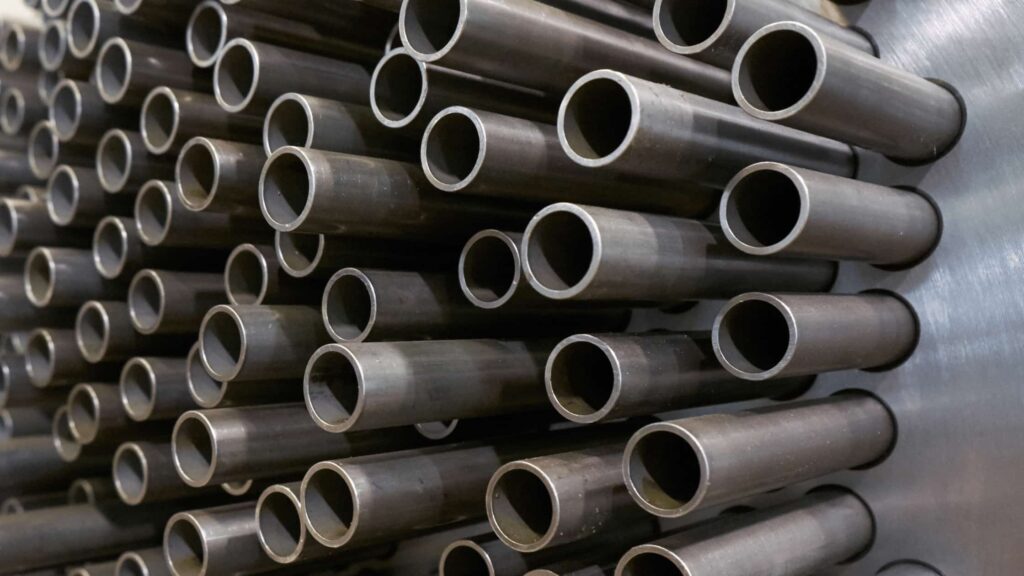
Carbon steel, composed primarily of carbon and iron, is a widely used material known for its low cost compared to other alloys. It comes in various grades based on carbon content, with higher carbon levels resulting in stronger and harder steel.
Carbon steel can be easily shaped and formed into different products, and its performance can be improved through heat treatment, which enhances its hardness, strength, and toughness. One notable property is its ferromagnetic nature, making it useful in motors and electrical appliances. Carbon steel is commonly used in the construction of electric motors, transformers, and generators due to its magnetic properties and cost-effectiveness.
Super Alloy
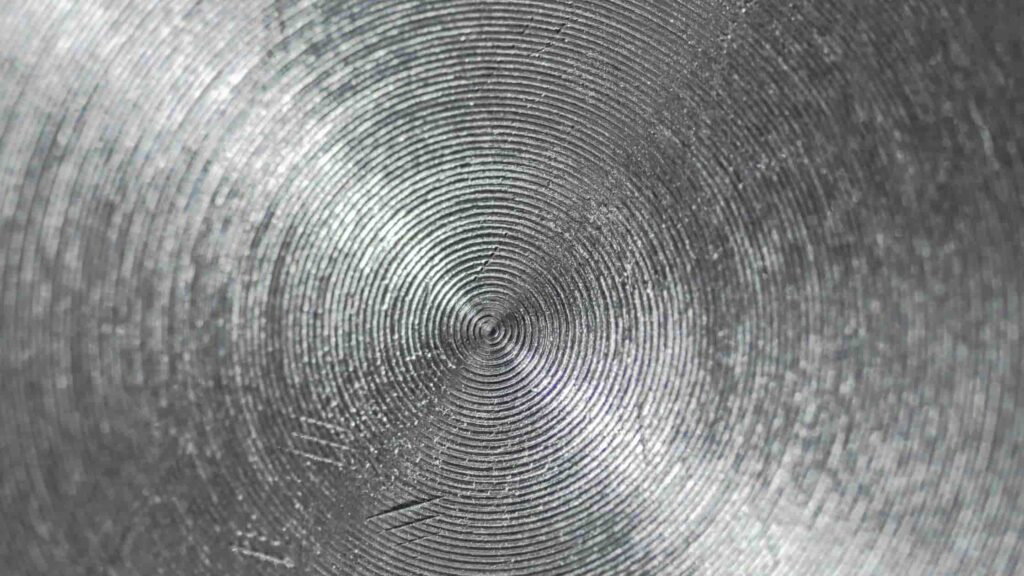
Super alloys, or high-performance alloys, particularly nickel-based and cobalt-based, exhibit exceptional mechanical strength, resistance to extreme temperatures, corrosion, oxidation, and wear. They retain their mechanical properties at temperatures exceeding 1000°C, making them indispensable in gas turbine engines, aerospace components, and nuclear reactors.
Super alloys also offer excellent corrosion resistance in aggressive chemicals, acids, and seawater, benefiting chemical processing plants, marine applications, and oil and gas exploration. Their wear resistance allows them to withstand abrasive materials and heavy mechanical loads, making them ideal for high-stress components like turbine blades and jet engine parts. Despite their higher cost compared to sheet metal, super alloys’ superior properties, reliability, and longevity make them a cost-effective choice for demanding applications.
Copper Alloy
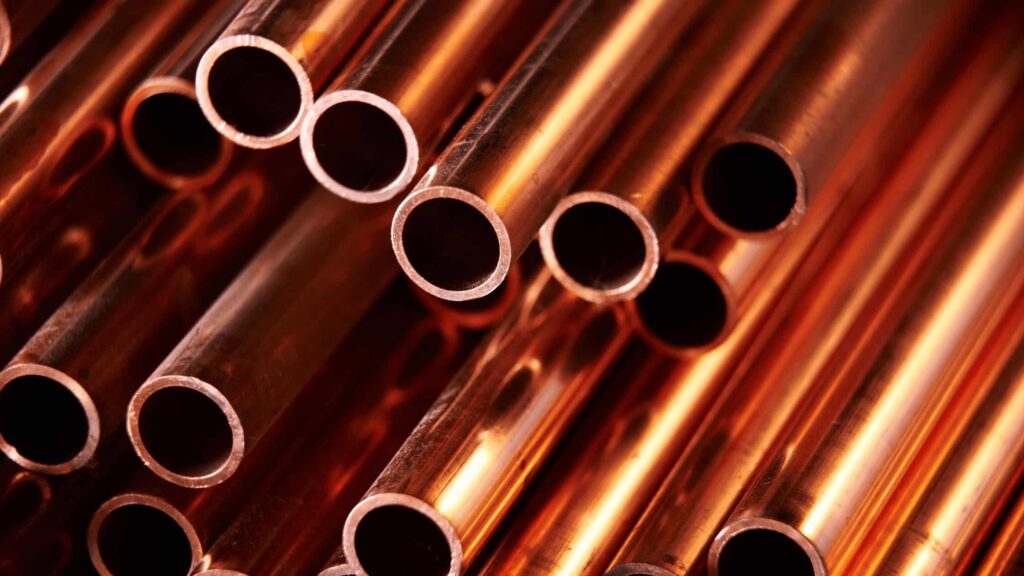
Copper alloys, created by mixing copper with other metals, offer superior strength, corrosion resistance, electrical conductivity, thermal conductivity, and good formability. Bronze and brass are two common types of copper-based alloys used in investment castings. Bronze, an alloy of copper and tin, offers high strength, corrosion resistance, and excellent thermal conductivity, making it suitable for musical instruments, statues, and fittings. Brass, an alloy of copper and zinc, is known for its attractive appearance and easy molding, making it popular for decorative items, plumbing fittings, and electrical connectors. Beryllium-copper, one of the strongest copper-based alloys, possesses exceptional corrosion resistance, high strength, hardness, and electrical conductivity, making it ideal for components in the aerospace and automotive industries subjected to harsh environments and high stress conditions.
Cast Iron
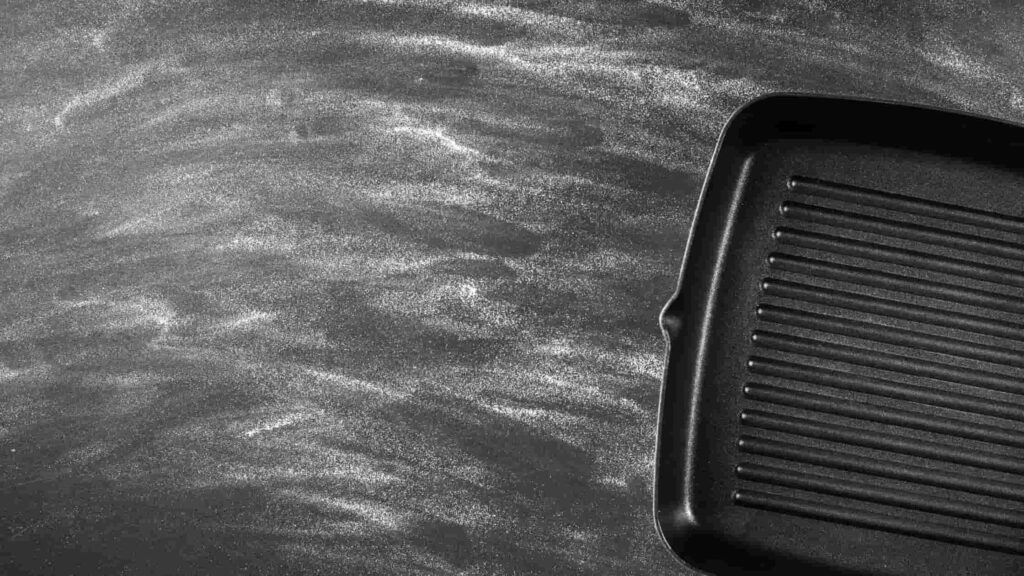
Cast iron is a strong and versatile material that is commonly used in various industries due to its unique characteristics. It is derived from iron and carbon, with a carbon content of at least 2%, making it harder and more brittle than steel. The production process of cast iron involves melting iron and adding carbon and other alloys to achieve the desired properties. This mixture is then poured into molds and allowed to cool and solidify.
One characteristic that makes cast iron desirable is its affordability and high accuracy. Iron castings, especially those made from gray iron and ductile iron, are known for their cost-effectiveness and precise dimensions. This makes them suitable for a wide range of applications, from automotive parts to appliances.
Gray iron, in particular, is easy to cast and has good damping properties, making it ideal for applications that require vibration resistance. On the other hand, ductile iron offers high strength, heat-resistance, and toughness. Its complex production process, which involves adding magnesium to the iron-carbon mixture, results in superior mechanical properties. However, this more intricate process leads to higher production costs compared to cast steel.
Key Takeaways
The selection of metal casting is a critical decision that significantly impacts the final product’s performance and cost-effectiveness. By carefully considering factors like the part’s function, desired mechanical properties, and compatibility with the investment casting process, manufacturers can ensure they choose the optimal ceramic material for their application. This not only guarantees the part meets its performance specifications but also minimizes the need for extensive post-processing, leading to improved production efficiency and tooling cost savings. Therefore, investing time and effort in material selection is a strategic step that unlocks the full potential of precision casting for a broad range of industries.
The Basics of Investment Casting: An Introduction
Investment casting, also referred to as precision casting, is a method for crafting detailed and high-quality metal parts with excellent accuracy and smooth finishes. It’s utilized in sectors such as aerospace, automotive, healthcare, and defense.
This technique offers multiple benefits, including the capability to fabricate complex and intricate designs that might be challenging or unachievable with other manufacturing processes. It also delivers high dimensional accuracy, as the mold faithfully reproduces the details of the die. Additionally, the method allows for fine surface finishes, reducing the need for further machining.
How Investment Casting Works
The investment casting process involves creating a wax pattern, encasing it with a ceramic material, and then heating it to remove the wax, leaving behind a mold. Molten metal is then poured into this mold, which solidifies to form the desired metal object.
Understanding the Step-by-Step Investment Casting Process
Step-by-Step Breakdown:
- Creating the Wax Pattern: The process begins by shaping a wax pattern of the part to be cast. This can be done by injecting wax into a metal mold or by sculpting the wax by hand. The wax model is then attached to wax sprues, which act like channels guiding the liquid metal during the casting.
- Building the Ceramic Shell:The wax pattern attached to sprues is dipped into a ceramic slurry followed by a coating of ceramic particles. This step is repeated multiple times to build a thick ceramic shell around the wax. Each layer must dry thoroughly before the next is applied.
- Melting Out the Wax:Once the ceramic shell is sufficiently thick, it is heated in a furnace. This step melts the wax inside the shell, which is then drained out, leaving a hollow ceramic mold that mirrors the original wax pattern.
- Pouring the Molten Metal:The ceramic mold is preheated to prepare for metal casting. Metal is melted in a crucible and then poured into the hot ceramic mold through the sprues.
- Removing the Ceramic Shell:After the metal has cooled and solidified, the ceramic mold is broken away, revealing the metal part. Sprues are cut off, and any remaining ceramic fragments are cleaned off the metal.
Further Steps to Perfect the Casting:
- Refining Wax Patterns:The accuracy of the wax patterns is crucial as they directly influence the final metal part’s details. Techniques like hand carving, using steel tools, or 3D printing are employed based on the complexity and quantity needed. Each method offers different benefits, from artisanal detail to consistent mass production.
- Setting Up the Wax Assembly:Wax patterns are mounted to a sprue and carefully arranged on a casting board to ensure stability and precise alignment during casting. This setup is crucial for maintaining design integrity and ensuring the molten metal flows correctly into each cavity.
- Forming the Mold Shell:The assembly is repeatedly dipped into a ceramic slurry and coated with sand stucco to form a strong, heat-resistant mold. Achieving the right thickness and strength of the shell is vital for withstanding the molten metal’s temperature.
- Wax Removal Techniques:Removing the wax can be done manually or using automated ejectors in the die, depending on the precision and delicacy required. This step is critical for maintaining the mold’s integrity and ensuring clean metal flow.
- Casting the Metal:Techniques such as gravity pouring, vacuum pouring, or pressure casting are chosen based on the part’s complexity and required precision. Each method has its advantages in achieving a defect-free cast.
- Final Touches on Castings:After breaking the mold, the castings are finalized by removing any excess material and refining the surfaces. This can involve various finishing techniques, depending on the metal and the part’s function.
What Is the Main Goal of Investment Casting?
It has been around for centuries and is used to create complex metal parts with great precision. The process of investment casting starts with a wax pattern, which is coated with ceramic to form a hollow mold after the wax is melted away. The main goal of lost-wax casting is to produce intricate, high-quality metal components that are tough or impossible to make with other methods. This casting process is favored in industries such as automotive, aerospace, defense, and jewelry for its ability to produce detailed and precise components.
If you are looking for a more in-depth explanation about the different materials that can be used in investment casting, check out our article about The Materials Behind Investment Casting.
Advantages of Investment Casting
Investment casting is great for making complex and detailed shapes that would be hard to make with other methods. It’s very accurate because the mold captures all the details of the wax model. It also makes parts with smooth surfaces, which means less work is needed to finish them. Some things made with this method include turbine blades, surgical tools, and jewelry.
Understanding the Disadvantages of Investment Casting Products
Despite its advantages, it’s important to be aware of the potential drawbacks of using this process. Here are some of the main disadvantages:
Size and Weight Limitations:
- Lost-wax casting has inherent limits on the size and weight of the products it can produce. The detailed nature of the process restricts the dimensions and mass that can be achieved effectively.
- This method is not ideal for manufacturing large parts or components that require substantial volumes of common material. For larger parts, alternative manufacturing methods might be necessary.
Extended Production Time:
- The process involves several intricate steps, including creating wax patterns, assembling ceramic shells, and multiple stages of heating and cooling. These steps contribute to a prolonged production cycle.
- This extended timeframe can be a significant disadvantage in industries where rapid time-to-market is crucial.
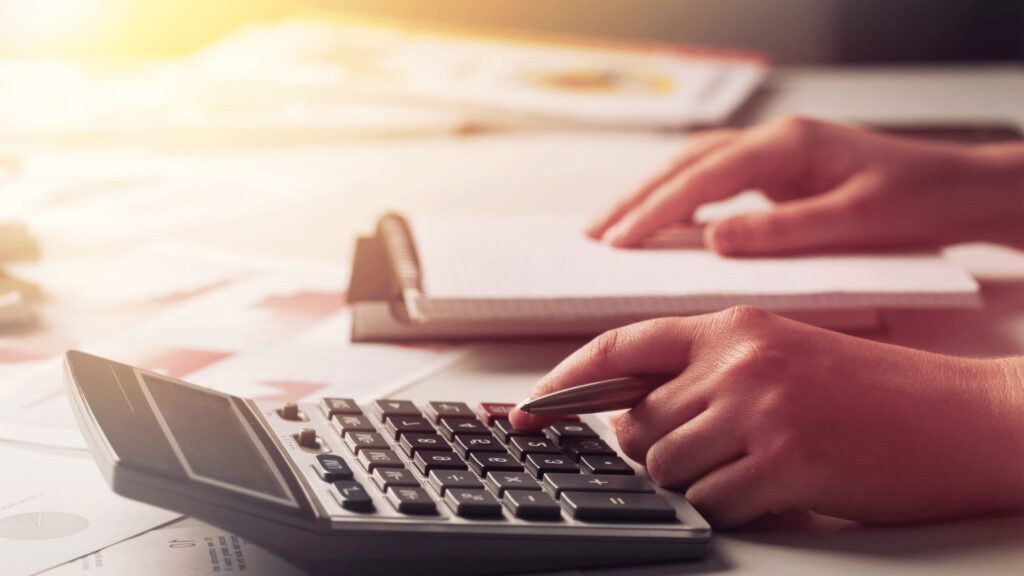
Assessing the Cost of Investment Casting
Although initially perceived as expensive, various aspects of the process contribute to its overall cost-effectiveness.
Initial Tooling Investment:
- It requires significant upfront investment in tooling, including the creation of wax patterns and ceramic molds. This precision work necessitates expertise, which adds to the initial costs.
- Despite these higher initial expenses, the durability of the molds allows them to be reused for multiple production runs, which lead to cost savings per unit over time.
Material Costs:
- The process uses a wide variety of metal alloys, such as stainless steel, aluminum, and titanium. Some of these materials are more expensive than others.
- Investment casting is efficient with materials, often using lightweight alternatives that help lower overall production costs by reducing material usage.
Efficiency and Waste Reduction:
- The method is notable for its efficiency and minimal waste production. Unlike other manufacturing techniques, investment casting produces complex parts without requiring extensive machining or secondary operations, which further cuts down on material and labor costs.
Detailed and Precise Outputs:
- The wax patterns used in investment casting allow for intricate designs and fine details, which are exact replicas in the final metal parts. The ceramic molds maintain the geometry of the wax patterns, ensuring the cast parts retain their complex features.
- This precision is challenging to achieve with other manufacturing processes, making investment casting particularly valuable for applications requiring high detail.
How Does Investment Casting Stand Out from Other Casting Methods?
Investment casting is unique compared to other types of casting like die casting and sand casting. The key difference is how the mold is made. In investment casting, a wax pattern of the final part is created first. This wax model is then covered with a ceramic material that hardens into a solid mold. Die casting, on the other hand, involves forcing molten metal under high pressure into a metal mold material. Sand casting uses a mixture of sand and a binder packed around a pattern to shape the mold cavity.
The precision and detail in investment casting are higher than in die casting and sand casting because the wax model can capture intricate details. This method is especially good for making thin-walled parts without any holes or gaps, achieving high accuracy.
Investment casting combines the benefits of detailed accuracy and reasonable costs, making it ideal for complex shapes and parts that need tight tolerances. It also allows for using various metal alloys, giving manufacturers flexibility in choosing materials.
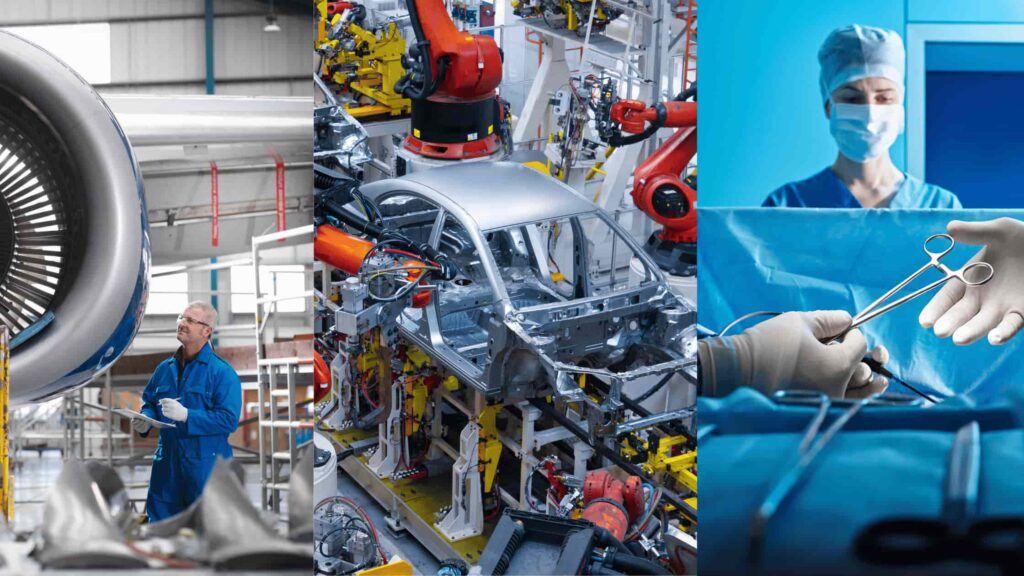
Which Industries Rely on Investment Casting?
Investment casting is popular across various sectors, including aerospace, automotive, and medical industries. In aerospace, it is used to make complex parts like turbine blades and engine components, offering excellent precision and minimal waste. Automotive manufacturers use investment casting for parts like cylinder heads and transmission components, benefiting from its ability to create lightweight and high-performance designs. The medical field also uses this technique for producing detailed implants and surgical tools, ensuring high precision and reliability essential for medical devices.
Exploring the Durability of Investment Casting Products
Precision casting products are renowned for their exceptional durability. This durability can be attributed to several factors including design, operational conditions, and material selection.
- Design Considerations: The durability of the products largely depends on their design. A well-thought-out design incorporates elements such as stress distribution, load-bearing capacity, and structural integrity. For instance, brass bearing surfaces in machinery are specifically designed to handle high loads and provide frictionless operation, which contributes to their longevity.
- Operational Limitations: The operating conditions that a product will face play a crucial role in determining its durability. Products are designed with these conditions in mind, ensuring they can withstand specific forces, vibrations, and environmental exposures. A prime example is automotive suspension knuckle joints, which are engineered to endure constant vibrations and harsh environmental conditions, thus enhancing their durability.
- Material Selection: Selecting appropriate materials is critical for the durability of investment casting products. The chosen materials must possess the necessary mechanical properties and corrosion resistance to ensure the product’s longevity. Stainless steel, for example, is often used in the process for its excellent strength, toughness, and resistance to corrosion.
- Additional Factors Contributing to Durability: It also allows for the creation of complex geometries, which can optimize the functionality and therefore the durability of the products. The process ensures precise dimensional accuracy, which is essential for the correct fit and alignment of components, enhancing both performance and durability. Furthermore, investment casting minimizes material wastage and facilitates the use of high-quality materials, both of which are instrumental in improving the structural integrity of the final product.
- Heat Resistance: The durability is also influenced by their ability to resist heat. This is dependent on the materials used; certain materials such as superalloys are chosen specifically for their exceptional heat resistance properties. These materials ensure that the products can operate effectively in high-temperature environments without degrading.
In summary, investment casting is a versatile process used in industrial applications for creating parts with different metals. It has size and cross-sectional limits, making it suitable for small to medium-sized parts. Correct product choice and strict process and quality control procedures are vital for successful investment casting.
Shakeout, Cleaning, and Finishing: Revealing the Cast
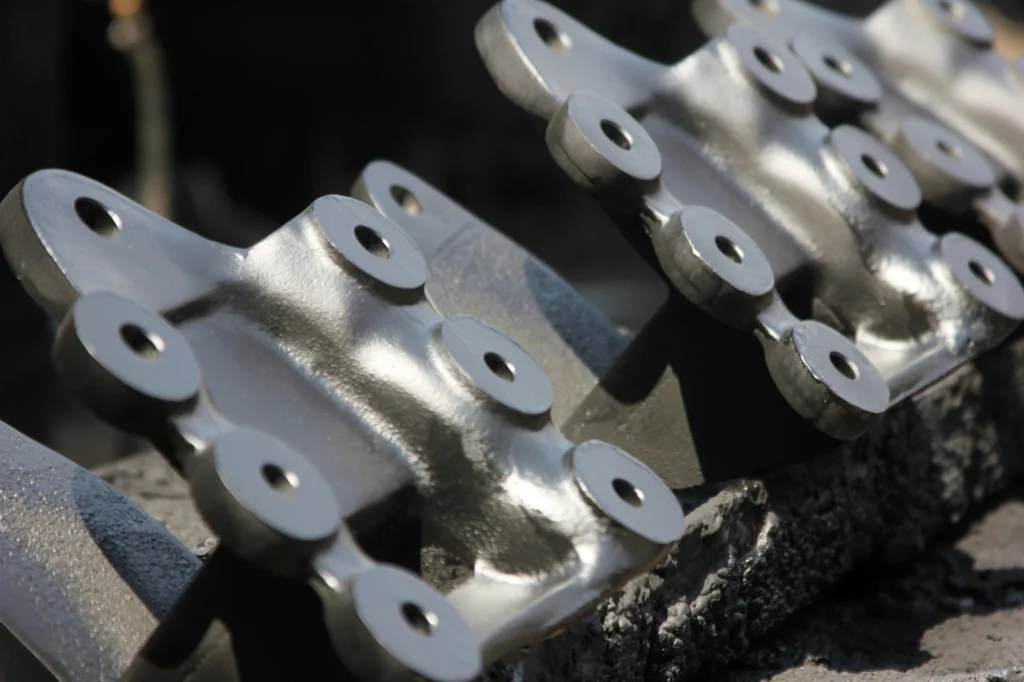
After the metal has cooled and solidified within the mold, the next phase is one of transformation, where the rough cast emerges from its sandy cocoon, ready to undergo the metamorphosis into a finished piece. This phase, comprising the shakeout, cleaning, and finishing processes, is crucial for revealing the cast’s true form and preparing it for its intended function. Let’s explore these steps that take the casting from a raw state to a polished final product.
The Shakeout Process: Separating the Cast Part from the Sand Mold
Shakeout marks the first crucial step in revealing a newly formed metal casting from its sand mold. This process involves carefully breaking apart the mold to uncover the casting, while ensuring the delicate new structure remains intact. The timing of shakeout is critical, as removing a casting too soon can expose it to rapid cooling, leading to undesirable effects such as embrittlement or microstructural changes. For example, in the case of ductile iron, premature exposure to air can alter its balance of pearlite, ferrite, or martensite structures, affecting the final material properties.
Foundries often rely on specific formulas or visual indicators to determine the optimal moment for shakeout. For instance, the greying of ductile iron is a common sign that it has cooled sufficiently to be safely removed.
Once the timing is right, the casting can be separated from the mold through various methods. In smaller foundries, this might involve manual tools such as tongs, where workers carefully lift the casting out of the mold as it crumbles away. In larger operations, automated systems like agitation or vibration tables are typically employed. These tables vigorously shake unflasked molds, causing the sand to detach from the casting and fall through perforated surfaces into collection bins. The reclaimed sand can then be treated and reused for future molds, contributing to sustainable foundry practices.
In some cases, sand molds include internal sand cores, which are used to create hollow sections within the casting. Removing these cores often requires additional methods, such as mechanical agitation, chemical treatment, or heat application, depending on the binder material used in the core construction. This ensures that all residual sand is dislodged and the casting is free from obstructions.
For cores made with chemical binders, additional measures may be necessary. For example, applying solutions of sodium or potassium hydroxide can dissolve certain binders effectively. Similarly, steam, cold water, or other thermal treatments can weaken the core material, facilitating its removal.
Once the core is sufficiently loosened, any remaining sand is typically cleared using a final mechanical step, such as an agitation table or pneumatic hammers. This ensures the casting is thoroughly free of residual material, preserving both the structural integrity and the precision of the design
By the end of the shakeout process, the casting is freed from its sandy cocoon, ready to move on to the next phase of cleaning and finishing. Each step requires a balance of efficiency and care to protect the structural integrity of the freshly unveiled piece.
Cleaning Operations: Fettling, Trimming, and Surface Enhancements
After the casting is removed from its mold, it must undergo a series of cleaning steps to remove residual sand, excess metal, and any imperfections caused during the casting process. This phase is traditionally referred to as fettling and is critical to preparing the casting for subsequent stages such as machining or final finishing.
Key Cleaning Steps in Fettling
- Trimming:
Excess metal, including gates, risers, and flash, is removed during trimming. These protrusions form where molten metal flows through the mold’s channels or leaks at the mold’s seams. Trimming typically involves mechanical cutting tools, such as saws or grinders, to ensure clean edges and a smooth surface. - Shot Blasting and Sandblasting:
To eliminate stubborn sand particles and refine the casting’s surface, abrasive blasting is employed.- Shot Blasting: Small steel balls or iron grit are propelled at high speed to remove scale, flash, and surface impurities. This process not only cleans but also slightly smoothens the surface.
- Sandblasting: Sand or other fine abrasives are directed at the surface under high pressure to dislodge debris and improve texture.
- Surface Treatments:
Additional treatments enhance the casting’s durability and appearance. These may include:- Acid Etching: Applied to clean the surface chemically and prepare it for further finishing.
- Shot Peening: Strengthens the metal by imparting compressive stress, enhancing fatigue resistance.
- Removing Flashing and Burrs:
Thin layers of excess metal, known as flashing, often form along the parting lines of the mold. Burrs, which are small, sharp protrusions, can also appear. These imperfections are carefully ground or filed away to ensure the casting is safe and functional. - Use of Tumblers and Automated Systems:
Castings may be placed in rotating tumblers or conveyors to be cleaned more thoroughly. In these systems, the components are agitated while abrasives like steel shot or air-blasting systems target hard-to-reach areas. - Final Fettling and Inspection:
After primary cleaning, fettling continues with detailed inspection and touch-ups. Pneumatic hammers or grinders may be used for intricate areas. At this stage, the casting is checked for surface flaws and dimensional accuracy.
Importance of Fettling in High-Quality Castings
Fettling transforms the rough, freshly cast metal into a refined and functional component. By removing imperfections and ensuring clean, smooth surfaces, this process plays a crucial role in meeting industry standards and preparing castings for specialized applications, whether in automotive, aerospace, or industrial machinery.
Incorporating Water Blasting in Cleaning Operations
In addition to traditional methods like shot blasting and tumbling, water blasting is another effective cleaning technique for castings. This method utilizes high-pressure water jets to clean the casting’s surface, often referred to as hydroblasting.
How Water Blasting Works
- Pressure Washing:
At its core, water blasting involves directing powerful streams of water at the casting. This pressure effectively removes residual sand, scale, and other surface impurities. - Abrasive-Enhanced Hydroblasting:
In advanced systems, abrasive materials like fine grit or beads are added to the water stream. This combination enhances the cleaning action, making it suitable for removing tougher contaminants and providing a finer surface finish. - Advantages:
- Eco-Friendly: Water blasting reduces the reliance on chemical cleaning agents and abrasives, making it an environmentally friendly option.
- Gentle on Castings: Unlike some abrasive methods, hydroblasting minimizes the risk of damaging delicate features or intricate details on the casting.
- Versatility: Suitable for a wide range of metals and casting types, from aluminum to steel.
- Applications:
Water blasting is often used in industries requiring high-quality finishes, such as aerospace or medical equipment manufacturing, where cleanliness and surface integrity are critical.
Role in the Cleaning Process
Water blasting can either be a primary cleaning step for delicate castings or a supplementary process after traditional methods like shot blasting. Following hydroblasting, castings may undergo inspection or additional surface treatments to achieve the desired finish.
By integrating modern water-blasting techniques, foundries can enhance efficiency, improve the casting’s surface quality, and reduce environmental impact.
Removing Gates and Risers
Gates and risers, essential features for directing molten metal through the mold, often leave behind excess material that must be removed during the cleaning process. Unlike smaller imperfections, these larger sections are too substantial to be dislodged through tumbling or air blasting.
Methods for Removal
- Oxy-Acetylene Torches:
High-temperature torches effectively cut through thick sections of excess metal, providing a precise and efficient removal method. - Chipping and Hammering:
Foundry workers may use tools like chipping hammers or sledges to physically break off gates and risers. This method is often employed for larger or more rugged castings. - Sawing:
Saws equipped with durable blades can trim excess stock, especially when precision is required. This is common for castings that demand detailed finishing. - Machining Integration:
In some cases, gates and risers are left for removal during the machining stage. This allows the removal process to be integrated with fine-tuning the part’s dimensions and surface quality, reducing redundancy in operations.
Recycling Removed Material
The metal trimmed from gates and risers is highly valuable. Unlike raw scrap, this material has already undergone purification during the casting process, as gases and impurities, such as paint or oil, are burned off in the furnace. This ensures the recycled metal is of superior quality, helping foundries maintain consistent material standards while minimizing waste.
Machining for Precision
Sand casting often produces surfaces that are rough and lack tight dimensional tolerances. To meet specific standards, additional machining is required.
Purpose of Machining
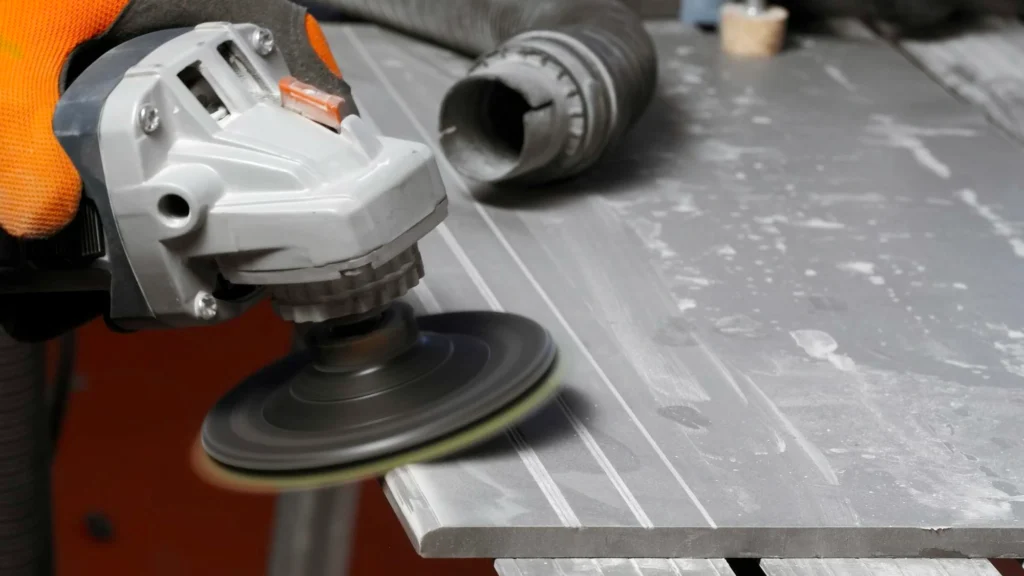
- Surface Finishing:
Machining creates a smoother surface, essential for parts that require aesthetic appeal or functional precision. - Dimensional Accuracy:
Tight tolerances are achieved through techniques like grinding, lathing, or milling. These methods ensure the part fits seamlessly with other components in assemblies. - Cost-Effective Design:
Since machining is costly, foundries aim to cast parts as close to the final shape as possible, minimizing the amount of material that requires machining. This reduces both time and expense while maintaining high-quality results.
By carefully removing gates and risers and employing machining only where necessary, foundries strike a balance between efficiency, cost, and precision, ensuring the castings meet exact specifications.
Finishing Touches: Machining, Painting, or Coating for Aesthetic and Functional Purposes
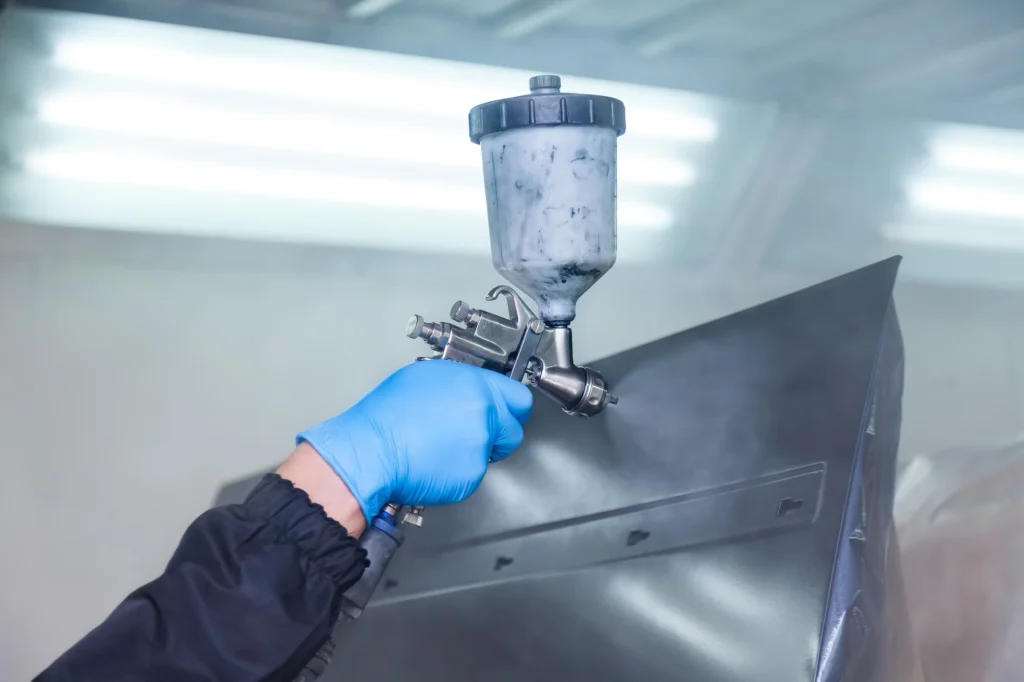
The final step in the casting process is finishing, where the cast part is refined to meet the precise specifications required for its intended use. This can involve a variety of processes, tailored to the demands of the final product:
- Machining: Precision machining is often necessary to achieve the exact dimensions and tolerances needed for the casting to function as designed. Processes like milling, drilling, and turning are used to refine the shape and size of the part.
- Painting or Coating: For aesthetic enhancement or to provide a protective layer against corrosion, castings may be painted or coated with various materials. This can range from simple paint applications to complex chemical coatings designed to withstand extreme environments.
- Quality Inspection: Finally, the casting undergoes a thorough inspection to ensure it meets all quality standards and specifications. This may include visual inspection, dimensional measurement, and non-destructive testing methods.
Real Life Examples
High-quality castings play a pivotal role in industries like automotive, where engine blocks and transmission housings must meet precise specifications. Similarly, in aerospace, turbine blades and structural components require exceptional durability and precision. For example, the aerospace industry often uses investment casting to produce lightweight yet robust components for jet engines.
Finishing technology is undergoing a revolution, with robotics and artificial intelligence transforming processes like machining and cleaning. For example, automated robotic grinders ensure precision in trimming and reduce manual labor. AI-powered systems are used for quality inspection, identifying defects with unmatched accuracy.
Sustainability in Foundries
Foundries today are adopting eco-friendly practices to reduce waste and improve efficiency. Sand reclamation systems, for instance, allow foundries to recycle up to 90% of their sand, reducing the environmental footprint. Additionally, advancements in closed-loop water systems minimize water waste, making foundries more sustainable.
Some foundries are also exploring green technologies like bio-based binders instead of synthetic chemicals for sand cores, lowering emissions and reducing reliance on fossil fuels.
Through the meticulous processes of shakeout, cleaning, and finishing, the casting is transformed from a rough, sand-encrusted object into a precise, polished component, ready to take its place in the world. This journey from mold to masterpiece underscores the blend of brute force and delicate precision that defines sand casting.
During the first production run, castings at this stage often serve as prototypes for clients or designers to assess for aesthetics and functionality. These prototypes provide crucial insight into the product’s viability and whether any design flaws need to be addressed.Align Manufacturing plays a key role ensuring that the final components meet the exact specifications and client expectations, making any necessary adjustments to improve the product before moving forward with full production.
Cooling and Solidification: A Delicate Transition
After the exhilarating moment of pouring molten metal into the mold, the sand casting process enters a phase of quiet transformation: cooling and solidification. This stage is pivotal, as the characteristics of the final casting are largely determined by the events that occur as the metal transitions from liquid to solid. Understanding the science of cooling curves and solidification patterns, alongside managing the factors that influence cooling rates, is essential in producing high-quality castings.
Explanation of Cooling Curves and Solidification Patterns
Cooling curves are graphical representations that show how the temperature of the metal changes over time as it cools and solidifies in the mold. Each metal or alloy has a unique cooling curve, influenced by its chemical composition, which dictates the rate at which it must be cooled to achieve desired properties. Solidification patterns, on the other hand, describe the manner in which the metal transforms from liquid to solid. These patterns can reveal insights into the potential for defects, the formation of different microstructures, and the overall quality of the cast.
Factors Affecting Cooling Rates and Their Impact on Casting Quality
Several factors influence how quickly and uniformly a casting cools, each affecting the casting's final characteristics:
- Mold Material: The thermal conductivity of the mold material plays a significant role. Sand molds, with relatively low thermal conductivity, cool the metal slower than metal molds, affecting the microstructure and mechanical properties of the casting.
- Casting Thickness: Thicker sections of a casting cool more slowly than thinner sections, leading to variations in microstructure and potential for defects such as shrinkage cavities.
- Temperature of the Molten Metal: The initial temperature of the molten metal when poured can influence the cooling rate; higher temperatures result in longer cooling times.
- Environmental Conditions: Ambient temperature and airflow around the mold can also affect cooling rates.
These factors can lead to a range of casting defects if not properly managed, including porosity, inclusions, and uneven mechanical properties.
Strategies to Control Cooling and Avoid Defects
To mitigate the risk of defects and ensure a high-quality casting, several strategies can be employed to control cooling rates:
- Chills: These are materials with high thermal conductivity that are placed in the mold to accelerate cooling in specific areas of the casting. Chills can be used to create more uniform cooling rates across the casting, preventing areas of localized shrinkage.
- Insulation: Conversely, insulating materials may be used to slow the cooling rate in certain sections of the casting to avoid thermal stresses and cracking. This is often necessary for castings with varying cross-sectional thicknesses.
- Controlled Pouring Temperature: Adjusting the temperature of the molten metal to the optimal pouring temperature for the specific alloy and casting size can help manage the cooling rate.
- Mold Design Adjustments: Modifying the design of the mold, including the thickness and placement of the sand, can help achieve more uniform cooling.
Cooling and solidification are not merely passive stages in the sand casting process; they are phases ripe with potential for influencing the final outcome. Through a deep understanding of the underlying principles and a strategic approach to managing cooling rates, foundry workers and artisans can significantly enhance the quality and integrity of their castings. This delicate transition, when navigated with skill and knowledge, marks the birth of a new metal form, ready to be revealed to the world.
FAQ:Everything You Need to Know
The cooling process directly impacts a casting's strength, structure, and durability. Faster cooling creates a finer grain structure, enhancing the metal's mechanical properties. Foundries adjust cooling rates by adding chills, using conductive sand types, or modifying mold designs to manage heat dissipation effectively.
A high-performing foundry goes beyond metal pouring by ensuring cleanliness throughout the process. It uses degassing techniques to remove hydrogen from aluminum melts, reducing porosity and improving strength. Applying flux treatment further purifies the molten metal, ensuring defect-free castings with reliable performance.
Foundries manipulate solidification by selecting appropriate mold materials and positioning cooling aids like chills. For example, zircon sand accelerates cooling due to its superior thermal conductivity. Feeder placement and mold design adjustments ensure an even cooling process, reducing internal stresses and shrinkage defects.
Maintaining the correct metal temperature prevents quality issues. Overheating may cause essential alloying elements to vaporize, weakening the final product. If the metal is too cool, incomplete fills or cold shuts can occur, leaving gaps in the casting. Temperature consistency ensures predictable and defect-free results.
Effective casting design minimizes common defects like gas bubbles, shrinkage cavities, and inclusions. A well-designed mold includes low-turbulence runner systems and rounded internal features to ensure smooth metal flow. These adjustments help produce structurally sound castings with fewer production failures.
Turbulence in the pouring process can lead to gas entrapment, inclusions, and reduced casting strength. To counteract this, foundries use controlled pouring methods with low-turbulence systems that reduce air pockets and ensure a smooth metal flow. This approach improves density and eliminates hidden flaws often detectable only through advanced inspections.